Topical drug delivery is one of the most challenging aspects of eye therapy. Eye drops are the most prevalent drug form because they are convenient and easy to apply by patients. However, conventional drug formulations are usually characterized by short retention time in the tear film, insufficient contact with epithelium, fast elimination, and difficulties in overcoming ocular tissue barriers. Not more than 5% of the total drug dose administered in eye drops reaches the interior ocular tissues. Drug-loaded nanoparticles/hydrogels do not enter cells via diffusion. The endocytosis pathway is related to the penetration of drug-loaded nanoparticles/hydrogels into the cell. The interactions between the nanoparticles and the cell membrane generate forces of different origins and lead to the membrane wrapping of the nanoparticles followed by cellular uptake.
1. Introduction
Most of the patients with eye diseases receive drug therapy. The bioavailability of the ophthalmic drugs after systemic administration is strongly restricted by the blood-ocular barrier system formed by two main barriers: the blood-aqueous barrier for the anterior segment and the blood-retinal barrier for the posterior eye segment (Figure 1). The former consists of the tight junctions of the non-pigmented epithelium of the ciliary body, iris tissues, and the iris blood vessels. This barrier limits the drug access to the anterior segment of the eye. The blood-retinal barrier is formed by junctions between retinal capillary endothelial cells and the tight junctions between retinal pigment epithelial cells [1].
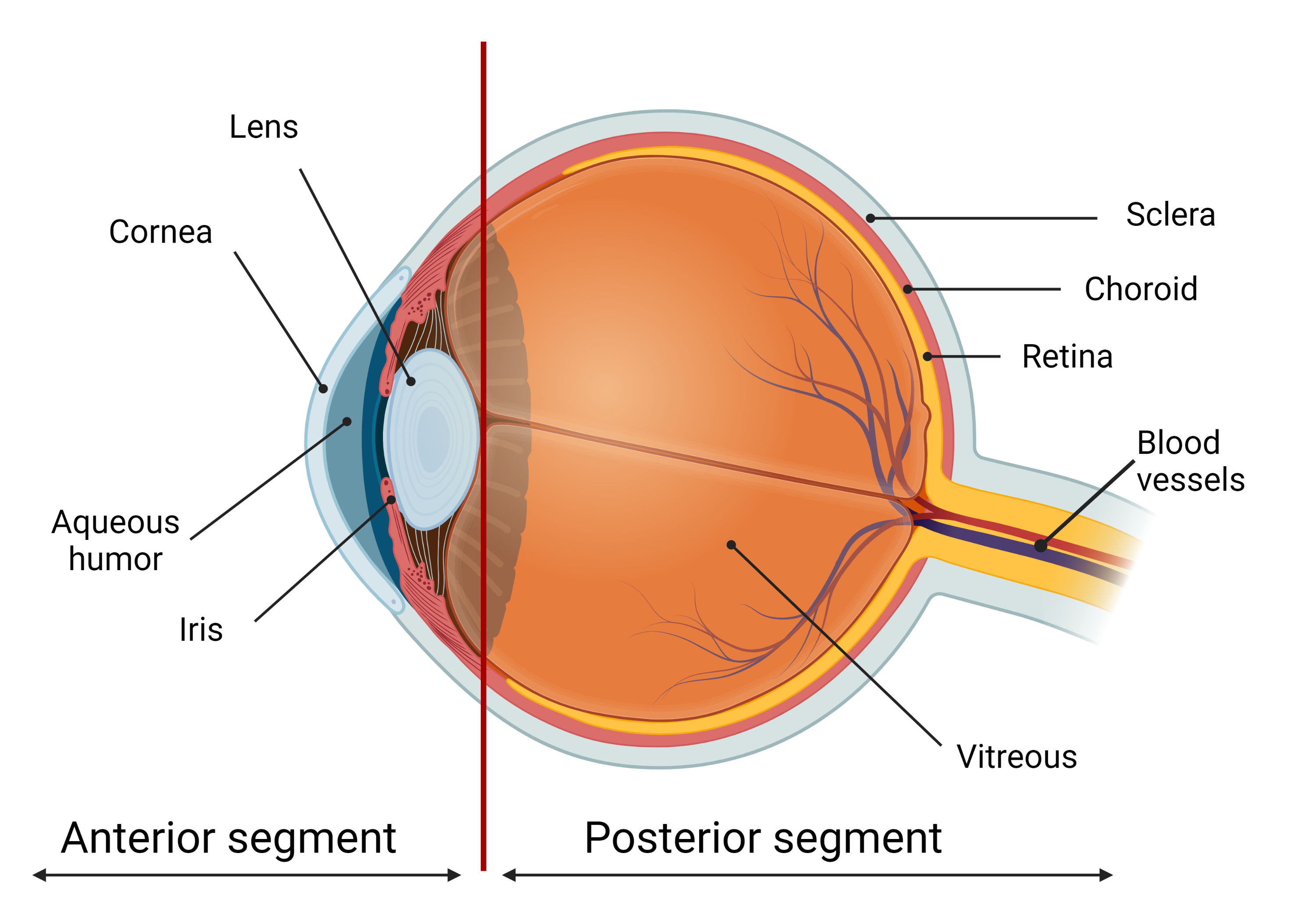
Figure 1. The scheme of the eye. The ocular globe can be conditionally divided into two parts: anterior segment and posterior segment. The anterior eye segment consists of cornea, conjunctiva, iris, ciliary body, lens, and aqueous humor, while sclera, choroid, retina, and vitreous body form the posterior segment. Created with BioRender.com.
The required amount of eye medication by local administration is significantly less than that by systemic administration. Local injections (subconjunctival or intravitreal) of the drugs are traumatic and have to be performed by medical personnel only. Topical administration of eye medications in the form of eye drops or gels is more favorable, as it can be easily performed by patients themselves. Eye drops are the most prevalent way of therapy for anterior eye segment diseases because they are convenient, demand less amount of the drug, and, consequently, are much less likely to cause side effects than after systemic administration. It is the major delivery route used for optimal drug absorption, especially for the treatment of anterior eye segment diseases. Eye drops represent about 90% of the marketed ophthalmic formulations [2][3][4][5][6][7].
However, only not more than 5% of the total dispensed dose of the drug administered in eye drops reaches the interior ocular tissues
[8]. Only ~30 µL of the liquid drug formulation can be applied to the eye due to the limited ocular surface area, and most of it is rapidly eliminated from the eye surface due to the tear turnover, blinking, and nasolacrimal drainage. Two minutes after instillation, about 60% of the drug was reported to be eliminated; after 8 min, the drug was diluted at 1/1000 and after 15–25 min, almost all an active ingredient was eliminated from the eye surface
[9].
Besides, tissue and fluid eye barriers restrict the influx of the active substance into the inner eye structures. The main route of topically administered agents into the anterior segment of the eye is via absorption through the cornea and conjunctiva with the subsequent transfer into the anterior chamber. The tear film on the cornea and conjunctiva covering the rest eye surface and the interior surface of the eyelids is the first permeability barrier limiting intraocular drug delivery. Tear film covers, hydrates, and protects the ocular surface, provides the eyelids sliding, and may delay the penetration of drugs into the eye. The human conjunctival sac can transitorily contain about 30 μL of tear fluid, and normal tear volume is estimated at roughly 7 μL. Tears have a high turnover rate (restoration time of 2–3 min), thus limiting ocular residence time for a drug
[10]. The tear film is a thin transparent fluid layer composed of three phases including an outer lipid phase, an intermediate aqueous phase, and an inner mucin layer (
Figure 2).
Figure 2. Schematic representation of the structure and composition of the cornea and the tear film. The cornea consists of the tear film, epithelium, Bowman’s membrane, stroma, Descemet’s membrane, and inner endothelium. The tear film consists of outer lipid phase, intermediate aqueous phase, and mucus. Created with BioRender.com.
The bulbar conjunctiva is a mucous membrane that covers the sclera, and the palpebral conjunctiva lines the inner side of the eyelids. Conjunctiva consists of the surface epithelium and inner stroma layer that comprise connective tissues with blood and lymphatic vessels. Conjunctiva is more permeable than the cornea, and its surface area is about 17 times larger than that of the cornea. Conjunctiva may provide a preferred route for the absorption of large and hydrophilic molecules
[11][12]. However, it is highly vascularized, and drugs penetrating the conjunctiva may be absorbed systematically directly from the conjunctival sac or the nasal cavity and reach general blood circulation rather than intraocular segments
[7][13]. It may cause significant drug loss into the systemic circulation thereby lowering its ocular bioavailability.
The principal problems in ocular drug delivery via topical administration are poor permeability of tissues and short residence time in the tear film. So, two main strategies have been followed to improve ocular bioavailability upon topical administration: (a) increasing pre-corneal retention time, and (b) enhancing corneal, scleral, and/or conjunctival drug permeability
[1]. To overcome the ocular drug delivery barriers and improve the drug bioavailability, various conventional and novel drug delivery systems have been developed such as emulsions, ointments, suspensions, aqueous gels, nanomicelles, nanoparticles, liposomes, dendrimers, implants, contact lenses, nanosuspensions, microneedles, in situ thermosensitive gels, etc. Among these, nanosize carriers are the most attractive, as they could enhance drug permeability across the blood-aqueous barrier and cornea, prolong drug contact time with ocular tissues, deliver drugs to a specific tissue in a controlled manner, protect drugs from degradation and metabolism to enhance drug stability, sustain drug release for a long time, diminish toxicity and side effects, maintain long shelf-life, while not needing to reconstitute or surgical removal
[14].
2. Polymeric Nanoparticles
Over the past decades, polymeric nanoparticles have become widespread in the field of drug delivery
[7][15][16][17][18][19][20][21][22]. Polymeric nanoparticles are defined as particles with a diameter of less than 1000 nm, consisting of various biodegradable polymeric materials. Nanoparticles need to be retained in the conjunctival sac, following topical instillation and subsequent release of the drug from the drug delivery system to achieve sustained drug release and long-term therapeutic activity. If the drug is washed out of the nanoparticles too quickly or too slowly, there would be either insufficiently sustained release of the drug or the concentration of the drug in tears might be too low to ensure the adequate healing effect. For effective retention, it is important to obtain nanoparticles from bioadhesive materials, otherwise, drug-containing nanoparticles would be eliminated from the precorneal site almost as quickly as a drug in solution.
As a basis for polymer nanoparticles, the following polymers are usually used: poly(D,L-lactide-co-glycolide) (PLGA); poly(ε-caprolactone) (PCL); polyacrylamide; Eudragit® (RS100 and RL100); polycyanoacrylate (PCA); polymethylmethacrylate; as well as natural polymers, e.g., chitosan, gelatin, sodium alginate, and albumin. Ophthalmic medications can be embedded directly into the nanoparticle matrix or attached to the surface of the nanoparticle.
Drug-loaded polymeric nanoparticles can represent nanospheres, where a drug is evenly distributed over the polymer matrix, or nanocapsules, where a drug is enclosed within the polymer shell. Besides, hydrophilic polymers such as polyethylene glycol (PEG) and polyvinylpyrrolidone (PVP) can bind to mucins by hydrogen bonds and/or electrostatic interactions. This ability could provide the increased contact time of the drug carrier with the ocular surface. A long period of contact is needed for the improved bioavailability of the ophthalmic drug and, in general, for the duration of the drug’s action and controlled release of the drug from the delivery system.
The first generation of polymeric nanoparticles from the acrylic polymer PCA demonstrated sustained release of an included drug, as well as retention of the drug on the corneal surface
[23][24]. However, after the discovery of PCA toxicity
[25], polyacrylate (second generation of nanoparticles) was considered to be more perspective
[26].
Then, it was demonstrated that nanoparticles consisting of polyesters (PCL and PLGA) are well tolerated by the eyes
[27], as well as nanoparticles consisting of hydrophilic polysaccharides, such as hyaluronic acid and chitosan
[28]. As the interaction of nanoparticles with the cornea is a key for long-term drug action, the third generation of nanoparticles has been developed which represent nanoparticles coated with a polymer having certain functional groups.
3. Polymeric Nanomicelles
Nanomicelles are composed of amphiphilic molecules (surfactants or block copolymers) that self-organize in an aqueous solution to form organized supramolecular structures [29][30][31]. During micellization, hydrophobic segments join to form a core region, while hydrophilic segments form a hydrophilic shell of micelles. Micelles of various sizes (10–1000 nm) and shapes can be obtained depending on the molecular weight of the polymer forming the core and crown-forming blocks. Micelle structure can be adapted to obtain unique properties considering delivery requirements, e.g., prolonged stability of micelles in tear fluid to increase the contact time with the cornea. A small particle size, ease of preparation, a high ability to encapsulate a drug made nanomicellar technology popular for ocular drug delivery [32][33]. Recently, nanomicellar approaches have been used with both polymer and surfactant to deliver locally small molecules as well as genes to the anterior segment of the eye [29][34].
In particular, most of the polymeric micelles used in drug delivery consist of amphiphilic di-block (hydrophilic-hydrophobic) polymers, tri-block (hydrophilic-hydrophobic-hydrophilic) polymers, graft (hydrophilic-hydrophobic), and ionic (hydrophilic-ionic) copolymers. For the majority of these systems, PEG is the primary hydrophilic segment. Also, the hydrophilic outer part of polymeric micelles can be composed of poly(ethylene oxide) (PEO), poly (acryloylmorpholine), poly(trimethylene carbonate), or poly(vinylpyrrolidone). Block copolymers such as PEO-poly(L-amino acids) provide an additional advantage to modify the properties of core-forming blocks. For example, the functional groups of the block are capable of chemical conjugation with a drug allowing efficient delivery of therapeutic doses. The hydrophobic core of polymeric micelles is usually made up of Pluronics (poly(ethylene oxide)-b-poly(propylene oxide)-b-poly(ethylene oxide)), poly(L-amino acids), such as poly(L-aspartate) and poly(L-glutamate), polyesters, such as poly(glycolic acid, PLA, PLGA, and PCL.
4. Inorganic Particles
Inorganic nanoparticles suitable for nanomedicine include gold
[35][36][37][38][39] and silver
[40][41] nanoparticles, cerium dioxide
[42][43], silica
[44][45], as well as inorganic salts
[46][47][48].
Some inorganic nanoparticles can be considered drugs themselves, as well as they can serve as carriers of medicinal compounds. Most often, the proposed particles consist of two regions: a core containing an inorganic component (for example, gold or silica) and a polymer cover for increased bioavailability
[36][37][45][49].
Gold nanoparticles themselves had a positive effect on endotoxin-induced uveitis in rats
[39]. Liposomes containing gold nanoparticles with flucytosine were suggested for the treatment of fungal eye infections
[38]. For the prevention of cataracts development, gold nanoparticles with N-acetylcarnosine stabilized with polyphenols were obtained
[50]. Gold nanoparticles with anti-glaucoma drug, timolol, were included in the contact lens
[37]. Also, gold nanoparticles with amfenac stabilized by poly(catechin) were proposed for the treatment of dry eye syndrome
[51].
Silver nanoparticles are known for their antimicrobial properties
[40][52][53], as well as their anti-angiogenic effect due to inhibition of the hypoxia factor HIF-1α in cells, which, in turn, leads to the suppression of the vascular endothelial growth factor VEGF-A function
[54]. It has been shown that a suspension of silver particles 15–50 nm in size has a powerful antioxidant effect, which could be potentially used to prevent the development of cataracts
[41]. However, it has been shown that silver nanoparticles are rather toxic
[55][56].
Nanoparticles based on cerium oxide are practically non-toxic and have a powerful antioxidant effect
[43][57]. Cerium oxide nanoparticles have been shown to mimic oxidoreductase enzymes by catalyzing the decomposition of organic substrates and reactive oxygen species. Moreover, the covering of 7.8 nm cerium oxide cores with two poly(sodium acrylate) and four poly(ethylene glycol) (PEG)-grafted copolymers with different terminal or anchoring end groups did not affect the superoxide dismutase-like activity but surprisingly improved peroxidase-like catalytic activities of cerium oxide nanoparticles
[58]. It has been shown that cerium nanoparticles penetrate well into the tissues of the eye; in particular, they remain in the retina for up to 1 year after a single intravitreal injection, without causing inflammatory processes
[57][59].
Modified silica nanoparticles have been used to include anti-glaucomatous drugs. Thereto amino-functionalized mesoporous silica particles containing brimonidine
[45] and gelatin-covered mesoporous silica nanoparticles containing pilocarpine
[60] were obtained. The resulting particles were shown to slowly release brimonidine in a sustained manner over 8 h. It is important that the particles were mucoadhesive, thus allowing the drug to reside in the tear film for up to 12 h because it adhered to the mucous layer. A decrease in IOP was observed even after 12 h
[45]. Gelatin-covered silica nanoparticles with pilocarpine were intracamerally administrated to the glaucomatous eyes. The in vivo studies showed the maintenance of normal IOP in the eyes with ocular hypertension for 21 days
[60].
Calcium carbonate particles could be obtained by simple mixing solutions containing calcium cations and carbonate anions. By varying the synthesis conditions, such as pH, temperature, and the presence of extraneous reagents, particles of different sizes and morphologies can be obtained
[61][62]. For example, when polyols or surfactants are added, exclusively vaterite particles were formed with a size of 1–2 µm
[62]. The inclusion of drugs in calcium carbonate particles can be carried out either by co-precipitation during the synthesis of the particles or by adsorption of the drug on the particles
[48][63][64]. It is worth noting that calcium carbonate particles possess mucoadhesive properties, which can improve their interaction with the ocular surface
[65].
5. In Situ Hydrogels as an Additional Carrier of Drug-Loaded Nanoparticles
The promising way to solve the problems of the rapid and extensive precorneal loss of the drug instilled in the eye caused by the drainage and the high tear fluid turnover, and to increase the contact time between the drug and the corneal surface is the use of stimuli-responsive hydrogels. They undergo sol-gel conversion «swell» in the presence of a specific biological stimulus (changes in temperature, pH, or electrolyte interaction) and can turn back into solution again when the trigger is removed. Thus, the most efficient drug delivery can be expected if drug-loaded nano-carriers are incorporated into the in situ gel. The sensitivity against the biological stimulus can be enhanced by using a combination of polymers that respond to multiple biological stimuli. Thus, the suspension of nanocarriers in the in situ gel can provide the advantages of both nano- and gel technologies
[66]. While the design of such formulations is one of the most challenging paths in the creation of new forms of drugs for ophthalmic delivery, these complex formulations might noticeably extend ocular residence time and improve the bioavailability of ophthalmic medications
[67][68][69].
Thermosensitive gels are the most studied of stimuli-sensitive polymer systems in drug delivery. The temperature of sol-gel transition is called the lower critical solution temperature (LCST). Below LCST, the system remains as a solution and above that temperature, the formulation transforms to a gel
[68][70][71][72]. So, the putative ophthalmic formulation should be a solution or, more correctly, colloid solution of nanoparticles or nanomicelles at room temperature. After instillation and contact with the eye surface, however, this formulation is expected to form gel containing nanoparticles/nanomicelles with a loaded ophthalmic agent.
Poloxamers or Pluronics
©—nonionic triblock copolymers composed of a central hydrophobic chain of poly(propylene oxide) flanked by two hydrophilic chains of poly(ethylene oxide)—represent one class of thermosensitive gels. These polymers and their combinations with other polymers have been extensively investigated for ocular drug delivery
[73][74][75][76].
6. Potential Ocular Nanomedicine
Over the past decade, ocular drug delivery systems for topical instillation have been extensively investigated. Many nanoformulations for the treatment of eyes have been developed and commercialized in the market (Figure 3). Some nanoformulations, such as drug-loading emulsions, are still slowly developing for treatment, even though drug-free nanoemulsion has been approved. The current ocular nanoformulations marketed products are Restasis® (nanoemulsion of cyclosporine A), Cyclokat® (cationic nanoemulsion of cyclosporine A), Cequa® (cyclosporine A ophthalmic nano micellar solution), DUREZOL® (difluprednate nanoemulsion), SYSTANE® (Propylene glycol-based nanoemulsion,) Lacrisek® (vitamin A and vitamin E liposomal spray), Artelac Rebalance® (vitamin B12 liposomal eye drops).
Figure 3. Schematic representation of ocular drug delivery systems for topical administration. Recently FDA approved and developed ocular drug systems presented. Created with BioRender.com
One of the important examples of FDA-approved drugs that have entered the market over the past decade is Inveltys
® (KPI-121 1.0%, Kala Pharmaceuticals). Inveltys
® is a nanosuspension of loteprednol etabonate (LE) delivered by a proprietary nanoparticle-based formulation referred to as mucus-penetrating particles. A preclinical study of the pharmacokinetics of LE-loaded nanoparticles showed a three-fold increase in C
max in the cornea, iris/ciliary body, aqueous humor, and retina compared to LE
[77]. In two randomized controlled clinical trials (and NCT02793817), 386 subjects were treated with KPI-121 1% and 325 were treated with placebo following cataract surgery. Combined data analysis showed that significantly more participants treated with KPI-121 vs. vehicle achieved complete resolution of anterior chamber cells at days 8 and 15 and complete clearing of ocular pain at days 4, 8, and 15. KPI-121 1% ophthalmic suspension was effective in resolving postoperative ocular inflammation and pain in patients following cataract surgery
[78].
7. Conclusions
Advances in the development of nanotechnology-based approaches to overcome the limits of the topical application of ocular drugs for the therapy of anterior segment eye diseases are quite impressive. The novel formulations were designed for better contact with the eye surface and increased residence time on the eye surface, better penetration into the eye, sustained drug release, and prolonged drug action. So, the effectiveness of these drugs could be substantially increased. Moreover, the dose and frequency of drug administration could be decreased by decreasing the local and systemic side effects of the drug. This is especially important for the treatment of chronic eye diseases, such as glaucoma. The advances in the usage of nanoformulations of drugs in ophthalmology are schematically presented in Figure 4.
Thus, nanotechnology demonstrated great potential in the creation of new formulations of ophthalmic drugs. However, future research needs more information on the dosage and administration regimen of these formulations, metabolic ways and ocular toxicity of all formulation components, their pharmacokinetics and pharmacodynamics, the release of the drug in different eye tissues, formulation stability, the influence of the method of the synthesis not only on physico-chemical properties of formulation but also on its physiological effect, the suitability of nanocarriers with respect to biodegradability and patient comfort, etc. It is important to understand and measure the influence of nanoparticulate parameters on their biological effects.
Figure 4. Schematic presentation of the advantages of the use of nanoformulations of ophthalmic drugs.