Surface-enhanced Raman spectroscopy (SERS) has become one of the most attractive analytical techniques due to its versatility and high sensitivity for a variety of analytes like dyes, food additives, pesticides, explosives, DNA, and other biomolecules at very low concentrations. Furthermore, SERS analysis possesses a broad range of properties: it is non-destructive, portable, easy to perform, highly sensitive, fast, cost-effective, and can be used when samples are present in water since the background signal is negligible. SERS substrates may consist of metallic nanoparticles, roughened metallic surfaces, or nanoengineered surfaces with metallic nanoparticles deposited on a solid support.
1. Surface-Enhanced Raman Spectroscopy (SERS)
In the late 1970s, it was discovered that Raman signals could be highly amplified by using a roughened silver substrate
[1]. This new technique was named surface-enhanced Raman spectroscopy (SERS) and has attracted the attention of researchers because it is able to detect a wide variety of analytes like dyes, food additives, pesticides, explosives, DNA, and other biomolecules in very low concentrations
[2]. Furthermore, SERS analysis possesses a broad range of properties: it is non-destructive, portable, easy to perform, highly sensitive, fast, cost-effective, and can be used when samples are present in water since the background signal is negligible
[3][4].
In SERS, the amplification of Raman signals can be estimated with the enhancement factor (
EF). The EF compares the ratio of the intensity of the strongest band in Raman spectroscopy (
IRS) to that in SERS (
ISERS), under identical experimental conditions (light source, light power, time of acquisition, etc.). It also considers the ratio of the number of adsorbed molecules in a given scattering volume for SERS (
NSERS) and Raman spectroscopy (
NRS) analysis respectively, as shown in the next equation
[5][6].
The previous equation is representative for each substrate.
Another parameter is the analytical enhancement factor (
AEF), in which the number of adsorbed molecules is replaced with the concentration of the analyte in the sample for Raman spectroscopy (
CRS) and SERS (
CSERS), respectively as written in the next Equation. This value, although useful for analytical applications cannot be used to compare different substrates because not all the molecules in the sample will contribute to the signal, only the ones close to the substrate
[5][6].
In order to understand how SERS works, it is imperative to remember that the intensity of the Raman scattering is proportional to the magnitude of the net change in the molecular polarization, and that this affects the induced dipole of the molecule μ, since it is expressed as the product of the polarizability α, and the strength of the electric field E.

Thus, for achieving stronger Raman signals, one of both parameters can be increased, which is possible by two mechanisms: (i) electromagnetic and (ii) chemical
[2][5][7]. The electromagnetic mechanism is believed to be the most important and arises due to the localized surface plasmon resonance (LSPR) of a nanostructured metallic substrate, an optical phenomenon in which light interacting with noble metallic nanoparticles, smaller than the incident wavelength, provokes the surface electrons to become polarized and oscillate collectively, which is known as a surface plasmon. During this process, the electrons will be displaced from the nuclei and then return to the original position, due to the Coulombic attraction, producing an oscillation of a particular frequency
[8][9][10]. Then, when the incident photons are in resonance with this oscillation, a strong electric field will be produced
[11]. Electromagnetic enhancement relies on Raman-active molecules being confined within these electromagnetic fields
[12].
The oscillation resonance frequency is dependent on the nature of the metal, it's size, shape, and surrounding medium; when resonance is achieved with the incident light, the Raman signal can be amplified with an EF up to 10
10 or 10
11, allowing the detection of single molecules. For silver, gold, and copper nanoparticles with sizes between 30 and 100 nanometers (nm), the plasmon resonance falls within the visible light range, presenting collective oscillations on rough substrates with large surface areas
[5][13][14][15].
However, the generated electric field is not uniformly distributed throughout the substrate. Instead, it is localized in narrow regions known as
hot spots which can be regions over the surface of single nanoparticles with irregular shapes and sharp corners, or gaps between neighbor nanoparticles, which are responsible for larger intensities
[16]. The subsequent diagram in
Figure 1, is a visual representation of how hot spots are located within nanoparticles.
Figure 1. Location of hot spots.
Consequently, moderate EFs are more frequent, because it is much more possible that the analytes are somewhere on the surface of the substrate rather than in between nanoparticles in hot spot regions
[17]. This has a direct impact on the reproducibility of SERS because the number of nanoparticles can vary greatly from one place to another and those substrates with non-homogeneous coverage will produce signals with different enhancements, depending on the position where the measurement takes place; areas where hot spots are found will produce large enhancements, whereas regions with single nanoparticles will not
[18][19]. Hence, substrates with high EF values are, in most cases, not reproducible. In fact, the usual relative standard deviation (RSD) of SERS measurements is between 15 and 20%, and when the system is averaged as a whole, the enhancement factor rarely exceeds 10
7 or 10
8 [13][20]. It is due to the location of hot spots that large enhancements are rare.
For this reason, even though the enhancement factor is important, it should not be seen as crucial for defining the performance of a SERS array. Aspects such as reproducibility, stability, detection limit, and selectivity have to be taken into consideration as well. As such, it becomes necessary to engineer nanostructured substrates with a greater number of hot spots arranged periodically to provide homogeneous enhancements. One strategy is to reduce the gap between nanoparticles and many reports claim that aggregation is desirable, but this should be evaluated carefully because it is difficult to control nanoparticle aggregation
[4][7][13][17][18].
On the other hand, the chemical mechanism is based on the formation of a charge-transfer complex between the analyte and the metallic surface. When these two are close enough, their wave functions will overlap and electrons can be transferred, changing the polarizability of the molecule and intensifying the Raman signals. For this reason, the enhancement will decrease rapidly as the distance between the analyte and the substrate increases, but they do not have to be in direct contact
[2][17][21]. However, this mechanism alone does not result in SERS, because its maximum contribution to the EF is of 2–3 orders of magnitude. It is the synergy between both mechanisms (electromagnetic and chemical) that makes it possible to obtain amplified Raman signals
[2][14][21].
To sum up, for obtaining good SERS performance, it should be remembered that SERS is wavelength dependent and it is imperative to use an appropriate light source that is in resonance with the surface plasmons of the metallic nanostructures . Other aspects that have to be considered are the optical, morphological, and adsorption properties of the substrate and its affinity to the analyte molecule
[5][7][22].
2. Photocatalytic process
One of the main advantages of using TiO2 as a support to deposit metal nanoparticles (MNPs) is that it allows, through the photocatalytic process, the self-cleaning of the substrate. In the photocatalytic mechanism, when irradiated with light of sufficient energy, the electrons (e−) of titanium dioxide are excited from the valence band (VB) to the conduction band (CB) and are trapped by the metallic nanoparticles (MNPs) leaving holes (h+) behind in the VB of TiO2. After this, the holes react with water to produce the hydroxyl radical (OH·), while the electrons react with molecular oxygen to form the superoxide radical (O2−), both of which can undergo further reactions to produce species that are powerful oxidants and can effectively degrade organic molecules [23][24]. The process is represented in Figure 2.
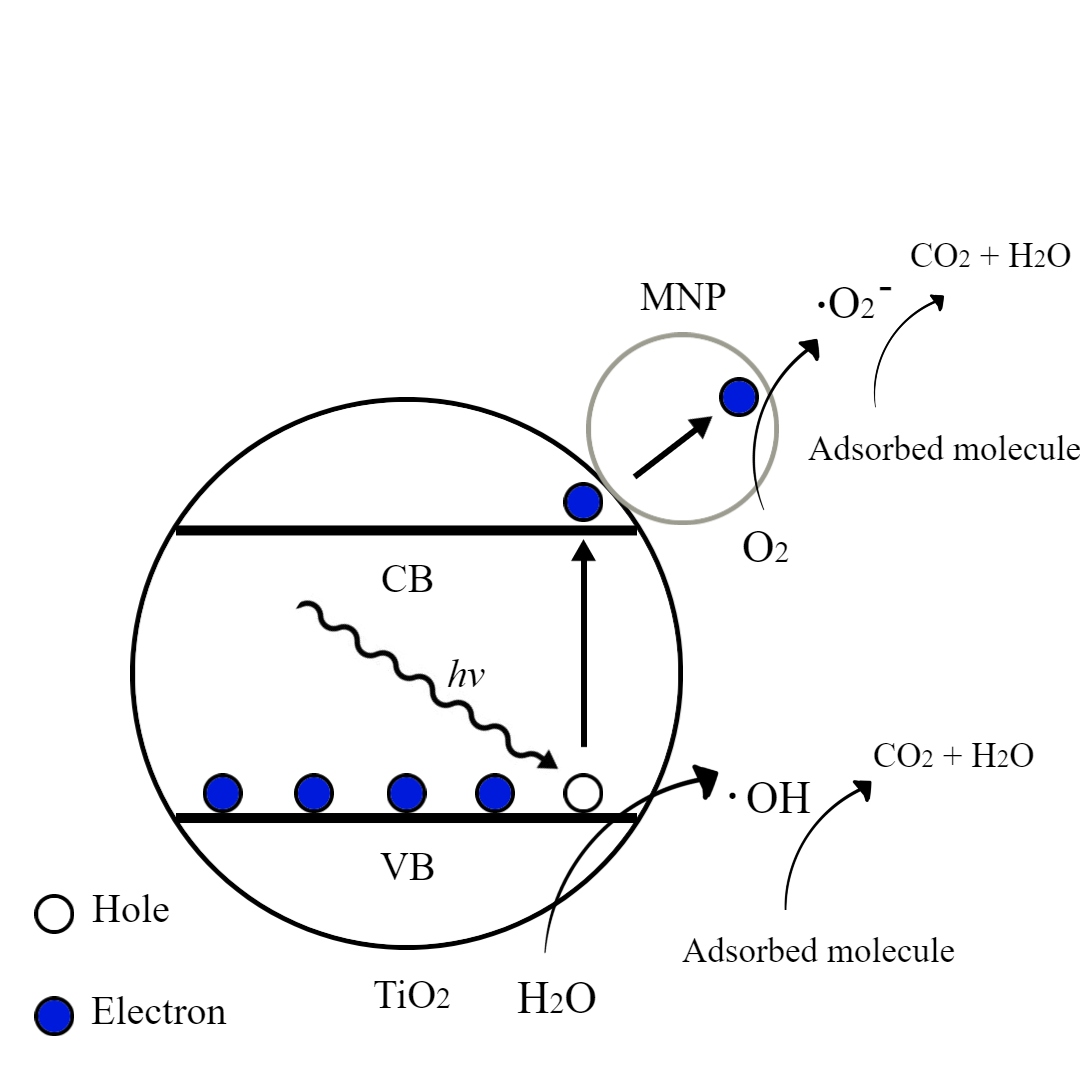
Figure 2. Photocatalytic mechanism to of TiO2/MNPs array to degrade adsorbed molecules.
Among the TiO2 nanostructures that have been studied as supports for MNPs, nanotube (NTs) arrays prepared by anodization have shown promising properties to build SERS platforms. Several features make them appropriate for this purpose: (a) the high surface area of TiO2 nanotubes allows to deposit a great number of MNPs, being able to have many hotspots [25]; (b) due to their photocatalytic properties can degrade the adsorbed molecules upon light irradiation to use the substrate for next measurements, achieving the desired recyclability [26]; (c) when the analytes are adsorbed on bare titanium dioxide nanotubes, no signal is observed, except for the band corresponding to titanium dioxide, as it has been reported by several works [11][25][27].
3. Silver Nanoparticles (Ag NPs)
For SERS applications, it was mentioned that metallic surfaces are required, copper, gold, and silver are mainly used; however, gold and silver are more stable than copper under ambient conditions and, compared to gold, silver is able to give higher signal enhancements
[28]. To this respect, Sun and colleagues reported an enhancement factor of 2.26 × 10
8 with a Ag NPs/TiO
2 NTs substrate to detect 2-mercaptobenzoxazole with a limit of detection close to 10
−9 M
[29]. On their part, Lamberti and his team reported a limit of detection (LOD) for Rhodamine 6G (RG6) in the range of 10
−14 M, defining this value as the concentration of the analyte at which the signal is three times greater than the standard deviation of the blank signal
[21][30]. What is more, silver nanoparticles are increasingly being used in optical sensors and in diagnostics due to their exceptional optical properties and because they represent a low-cost and scalable option for SERS purposes
[31][32].
Silver nanoparticles are the most used substrates since they have a broad surface plasmon resonance, which goes from the visible to the near-infrared regions, and because they are stable and easy to prepare
[33]. Various techniques are reported to obtain Ag NPs on solid substrates for SERS applications including thermal evaporation, ion sputtering, electrochemical, wet chemical, vapor-phase synthesis, and photoreduction
[28][34].
Silver Nanoparticle Deposition.
Notably, wet chemical techniques have been widely used for obtaining Ag NPs of different shapes and sizes by using capping ligands like trisodium citrate and poly(vinylpyrrolidone) (PVP) but these species cause interference in SERS measurements. In contrast, by using photoreduction and sputtering deposition methods it is possible to obtain clean substrates that are free of impurities and upon varying the synthesis conditions, it is possible to obtain nanoparticles of different size and size distribution
[33][35][36].
Additionally, it is possible to vary the amount of silver deposit onto titanium dioxide nanotubes to study the effect on the SERS signals, In fact, there are some reports in the literature where sputtering is used to deposit silver nanoparticles onto TiO
2 NTs prepared by anodization. In several reports, the sputtering is performed under a pressure of 3 × 10
−3 Pa and the silver deposit usually varies from 0.01 mg/cm
2 to 0.2 mg/cm
2, and it is mentioned that, generally, the signals for probe molecules such as pyridine or mercaptobenzoic acid, are either almost equal or more intense than those of a reference electrochemically-roughened silver substrate, whose enhancement factor is in the range 10
6–10
7, demonstrating that the superior activity makes these substrates promising for SERS applications
[11][36][37][38]
Plus, it is possible to modulate the optical properties of the metallic nanoparticles regarding the shape, position, and width of the localized surface plasmon resonance peak, by adjusting the synthesis conditions
[39][40]. The reason being is that the LSPR peak is very sensitive to any change in the environment of the substrate, and by modifying the size or shape of the nanoparticles it is possible to shift it
[40].
In fact, He and colleagues observed this phenomenon and used it to investigate how the SERS activity towards RG6 is affected by varying the size of Ag NPs and its LSPR peak. They deposited silver nanoparticles onto a functionalized glass substrate to obtain sizes from 35 to 65 nm, finding that the particles were either alone or formed clusters. In addition, as the particle diameter increased, the homogeneity of the size distribution decreased, as well as the surface coverage. Despite this, with UV-visible spectroscopy, it was seen that with larger nanoparticle size, an absorption band appeared above 600 nm that red-shifted gradually, arising from dimers and other particle aggregates. Then, SERS measurements were performed using an excitation laser with a wavelength of 633 nm; it was found that even though the surface coverage diminished, the highest enhancements were obtained with the biggest nanoparticles, which was attributed to large size distributions that allowed to obtain various hot spots configurations and because the LSPR band was in resonance with the used excitation wavelength
[31].
Finally, the silver nanoparticle–titanium dioxide nanotubes are able to degrade the adsorbed molecules upon UV irradiation, being an excellent choice for SERS because it allows multiple uses, eliminating the one-time use drawback of some substrates
[34]. Moreover, Sun and co-workers report that photocatalytic degradation of the adsorbed molecules has no impact on the morphology of the substrate, as evidenced by XPS measurements. They found that the adsorbed molecules were degraded onto smaller ones (including carbon dioxide and water), which can be easily removed by washing the substrate
[29]. Additionally, Chong and co-workers observed that once that the Ag/TiO
2 NTs array is cleaned upon UV irradiation, it is possible to achieve reproducible signals on further SERS measurements, as performed on R6G molecules, demonstrating the reusability
[34].
4. Gold Nanoparticles (Au NPs)
Gold nanoparticles (Au NPs) have been extensively studied as SERS substrates as they can be easily prepared either from chemical routes as the reduction of HAuCl
4 with a proper reducing agent (sodium borohydride, trisodium citrate, ethylendiaminetetracetic acid, formaldehyde, hydrazine, hydroxylamine, polyols, oxalic acid, sugars, etc.), through biological methods that make use of plant extracts (
M. sativa,
A. vera,
C. gossypium, among several others) or by physical methods such as laser ablation, vacuum sputtering or condensation methods
[5][41][42][43][44]. They also present very good biocompatibility, low reactivity, and can be easily modified on their surfaces by chemical methods, aside from exhibiting a strong plasmonic response, which is strongly tied to changes in their surroundings and to the electronic structure of substrates where they are deposited
[45]. Due to all those characteristics, Au NPs are of potential use in the development of biosensors, medical imaging, catalysis, among other applications
[46]. Au NPs can be easily deposited on different types of materials such as glass slides, mesoporous silica, tin oxide thin films, silicon wafers, aluminum foil, copper, polymer films, paper, plastic, etc., using different methods such as centrifugation/sedimentation, ultrasonic dispersion, thermal annealing, wet immersion deposition, chemical spray pyrolysis, convective assembly through Coulombic interactions, sputtering, silane-immobilization, electrophoretic deposition, precision photoreduction, among several others
[35][47][48][49][50][51]
Gold Nanoparticle Deposition on TiO2 NTs
Nanocomposites formed by incorporating noble metal nanoparticles into photocatalytic materials (plasmonic photocatalyst nanocomposites) have attracted the attention of several groups
[52]. The formation of Au NPs composites with different types of semiconductor materials (ZnO, CeO
2, TiO
2, Fe
3O
4) has been explored as they can present improved sensing performance due to charge transfer from the metal oxide to the Au NPs, which can be used to modulate the electrochemical response, although it can also be used for designing SERS detectors. There are several reports that describe how the deposition of Au NPs on TiO
2 NTs substrates enhances the surface electron interactions by plasmon resonance, improving the SERS response. Composites prepared by depositing TiO
2 decorated with Au NPs on reduced graphene oxide nanosheets (TiO
2-Au-rGO) have been also explored, which present high sensitivity for the detection of R6G up to 1.2 × 10
−10 M
[53].
Furthermore, as the TiO
2 substrate keeps its photocatalytic properties, it can self-clean after UV-radiation exposition, allowing recyclability. Deposition of Au NPs on porous photoactive TiO
2 nanostructures has been explored as a way to increase surface area and induce plasmonic coupling among adjacent Au NPs. Photocatalytic degradation can also be followed in situ in these types of systems by using Raman microspectroscopy as recently reviewed
[3]. For example, a recyclable SERS substrate for multifold organic pollutants detection based on Au-coated TiO
2 nanotube arrays was reported by Li and co-workers were Au NPs were assembled on the TiO
2 nanotube arrays by either the photo-deposition or the hydrothermal method. SERS spectra measurements on aqueous R6G solutions up to the 10
−6 M concentration, as well as on solutions containing 4-chlorophenol (4-CP), persistent organic pollutants (POP), dichlorophenoxyacetic acid (2,4-D), and methyl-parathion (MP) in ethanol or methanol were performed. The nanocomposite arrays were highly sensitive, stable, reproducible, and recyclable, as after the organic pollutants are detected by the SERS effect, they can be easily degraded into clean inorganic molecules by UV irradiation of the substrates
[54].
Bimetallic systems, using Au NPs as core and an Ag shell, have been also explored due to the potential synergistic electromagnetic coupling between the two metals. The group of Wang et al. reported a recyclable microfluidic Au@Ag/TiO
2 NTs SERS chip prepared by the in situ growth of Au@Ag NPs in the microchannels of the TiO
2 NTs
[55]. Evaluation of the SERS response towards R6G achieved very good sensitivity (up to 10
−10 M) and repeatability; charge transfer from TiO
2, the Au@Au plasmon, and the adsorbed molecules contained in the 3D NTs structure enhanced the SERS response. The shape of the bimetallic nanoparticles affects the SERS response; in another work, core-shell Au@Ag nanocubes were prepared by the epitaxial method, showing a strong plasmonic coupling among the Au core and the Ag shell in the intra-junction space, which produces a stronger SERS enhancement in comparison to pure Ag nanocubes
[56]. That work suggests that designing bimetallic nanoparticles with controlled morphologies, different shapes and complexities may be useful to design better systems for sensor or imaging applications.
5. Platinum Nanoparticles (Pt NPs)
Platinum nanoparticles are perhaps mostly known because of their catalytic behavior. Their large surface areas enable them to have outstanding performances that are exploited in the chemical industry for catalysis
[57][58]. Although, since these nanostructures have free electrons, surface plasmon resonance effects can be observed as well, and the activity in SERS has been demonstrated although rarely reported
[57][58][59].
Platinum Nanoparticle Deposition on TiO2 NTs
Similar to silver nanoparticles, it is possible to modulate the properties of Pt NPs by controlling the synthesis conditions
[57]. As an example, Cai and co-workers modified an anatase-phase TiO
2 NTs substrate with polydopamine (PDA), whose functional groups served as anchors for platinum nanoparticles. Then, different PDA/TiO
2 NTs substrates were submerged in solutions of H
2PtCl
6 of diverse concentrations, ranging from 0.1 × 10
−3 to 0.8 × 10
−3 M, under stirring and at 90 °C for 3 h. Upon SEM images it was found that, at the lowest concentration precursor, a small amount of nanoparticles were present on the surface of the nanotubes; by increasing the concentration to 0.4 × 10
−3 M, it was possible to observe clusters of aggregated nanoparticles and at 0.8 × 10
−3 M the nanoparticles aggregated and nearly blocked the nanotubes open ends. Thus, the density of the nanoparticles on the surface of the nanotubes increased with the precursor concentration. This had an impact on the SERS spectra of R6G, whose signals increased with the amount of nanoparticles until obtaining a maximum enhancement factor of 4.3 × 10
4 at a precursor concentration of 0.4 × 10
−3 M, attributed to the LSPR effect of the nanoparticles and the charge transfer of TiO
2 [59].
Thus, it is demonstrated that other noble metals can be used for SERS applications but a disadvantage is that they do not produce as large enhancements as silver, gold, or copper. Actually, the enhancements factors obtained with other transition metals are estimated to be between 1 and 4 orders of magnitude
[60]. Nevertheless, these arrays also show self-cleaning ability because Pt NPs are able to degrade the adsorbed species and trap the photogenerated electrons from TiO
2 and impede the recombination
[61]
In fact, Cai and co-workers were able to demonstrate the separation of photogenerated electron-hole pairs by measuring the current of the different substrates under light irradiation and at an applied potential of 0.26 V (vs. Ag/AgCl). Bare nanotubes had the lowest photocurrent, with a value of 0.003 mA/cm
2 due to rapid electron-hole recombination. In contrast, when Pt NPs were present, the photocurrents increased greatly with values of 0.038, 0.046, 0.068, and 0.054 mA/cm
2 for the substrates submerged in the H
2PtCl
6 solutions at concentrations 0.1 × 10
−3, 0.2 × 10
−3, 0.4 × 10
−3, and 0.8 × 10
−3 M, respectively, demonstrating that platinum nanoparticles are able to hinder the recombination of photogenerated species. This, in turn, served for the recyclability purpose because, upon UV irradiation, the signals from R6G nearly vanished after 150 min. Therefore, the development of nanoplatforms of platinum nanoparticles and titanium dioxide nanotubes relies on the interest of building SERS substrates that can be reused
[59].