Modified pectin (MP) is a bioactive complex polysaccharide that is broken down into smaller fragments of units and used as an oral dietary supplement for cell proliferation. MP is safe and non-toxic with promising therapeutic properties with regard to targeting galectin-3 (GAL-3) toward the prevention and inhibition of viral infections through the modulation of the immune response and anti-inflammatory cytokine effects. This effect of MP as a GAL-3 antagonism, which has shown benefits in preclinical and clinical models, may be of relevance to the progression of the novel severe acute respiratory syndrome coronavirus-2 (SARS-CoV-2) in coronavirus disease 2019 patients. The outbreak of emerging infectious diseases continues to pose a threat to human health. Further to the circulation of multiple variants of SARS-CoV-2, an effective and alternative therapeutic approach to combat it has become pertinent. The use of MP as a GAL-3 inhibitor could serve as an antiviral agent blocking against the SARS-CoV-2-binding spike protein.
1. Introduction
SARS-CoV-2 belongs to enveloped RNA viruses, subgenus betacoronavirus of the family coronaviridae
[1][2][3]. SARS-CoV-2 is made up of four primary structural proteins: homotrimer spike (S) glycoprotein, small envelope (E) glycoprotein, membrane (M) glycoprotein and nucleocapsid (N) protein as well as a few ancillary proteins
[3]. In coronavirus, the spike proteins (with a distinctive “corona” crown-like shape on the virion surface) have two subunits, the S1 and S2 glycoproteins. S1 has an N-terminal domain (NTD) and a C-terminal domain (CTD), which facilitate the host adhesion binding to the receptor. S2 has a CTD responsible for the fusion of the viral and cellular membranes and the entrance into the cells by attracting angiotensin-converting enzyme 2 (ACE-2)
[4][5].
The recent literature has indicated a COVID-19 treatment with a GAL-3-targeted therapy linking the spike proteins of coronaviruses, with human GAL-3 having a similar protein structure
[6][7][8]. This involves the use of the GAL-3 inhibitor as an antiviral agent blocking against the SARS-CoV-2-binding spike protein. However, there is still more to explore and understand about this promising GAL-3 target therapy route. Recently, attention has been paid to the potential involvement of pectin as a bioactive complex polysaccharide, which when broken down into smaller fragments is referred to as modified pectin (MP). The antagonistic activity of MP against GAL-3 has been of great interest in the majority of the preclinical and clinical reports in the prevention of and reduction in cancer
[9][10][11][12][13][14] as well as fibrotic, renal injury and cardiovascular diseases
[15][16][17][18] and inflammatory and immune functions
[19][20][21][22].
The effects of MP are the subject of new and interesting research with evidence indicating that galactan-rich tiny molecular weight pectin fragments can bind to the carbohydrate recognition domain (CRD) on the pro-metastatic protein, GAL-3. This hinders GAL-3 from interacting with other proteins and peptides, limiting its capacity to stimulate cell adhesion, migration, angiogenesis, tumorigenesis and apoptosis
[23][24][25]. This suggests that MP through the inhibition of GAL-3 could be used in a potentially safe and non-toxic method to prevent or reduce the viral adhesion and viral-associated inflammatory responses targeting a therapeutic approach
[8][26].
2. Potential Role of Modified Pectin Binding Galectin-3 in SARS-CoV-2 Infections
Studies have shown the potential role of industrial pectin in exploring its antiviral activity. In most MCP research, GAL-3 plays several prominent roles which influence its bioactivity by inducing extracellular functions such as the interaction between cells and inflammation. The relevance of the effect of MP through GAL-3 antagonism to the progression of SARS-CoV-2 in COVID-19 patients can be extrapolated from the perspective of (i) MP and GAL-3 binding as a mediator for viral adhesion in the virus infection mechanism through the viral spike protein, given that the N-terminal domain of SARS-CoV-2 evolves from a galectin origin, (ii) MP and GAL-3 response to inflammation and macrophage driving the cytokine storm in severe SARS-CoV-2 cases.
2.1. Modified Pectin and Galectin-3 Binding against a Viral Adhesion
GAL-3 has been identified as a binding mediator that aids viral attachment. In SARS-CoV-2 infection, the glycosylation of the outer membrane spike glycoprotein causes the interaction of the viral protein with the cell receptors and adhesion factors, including ACE-2. It was hypothesized that the unique N- and O-linked glycosylation sites of the S1-NTD spike glycoprotein in SARS-CoV-2 may interact with immunoregulatory factors
[27]. This suggests that GAL-3 plays an important role in SARS-CoV-2 host interaction
[8]. NTD and CTD domains can function as receptor-binding domains (RBDs). The S1-NTDs are in charge of sugar-binding, recognizing both N-acetylneuraminic acid (Neu5Ac) and N-glycolylneuraminic acid (Neu5Gc) sugar receptors
[28][29][30] and the S1-CTDs detect ACE-2 protein receptor
[31][32]. It is noteworthy that a recent study reveals a dual interaction of S1-NTD binding to the sugar co-receptor, Neu5Ac
[28] and the ACE-2 receptor in SARS-CoV-2 infection. The negatively charged -COOH terminal of the 9-O-acetyl-Neu5Ac is positioned for interaction with available sugar or protein molecules
[33][34].
SARS-CoV-2 may be able to recognize Neu5Ac co-receptors besides the ACE-2 by acquiring the GTNGTKR motif on the S1-NTD
[35]. In extreme cases of SARS-CoV-2, a new type of sialic acid-linked ganglioside-binding domain was discovered at the S protein’s N-terminal domain
[35]. It is therefore noteworthy that SARS-CoV-2 binds to Neu5Ac, GAL-3, GTNGTKR motif, and sialic acids-linked ganglioside could contribute to the greater infectivity of the virus compared to the SARS-CoV
[28][35][36]. Similarly, the abundance of Neu5Ac and GAL-3 receptors in the human body particularly at the nasopharynx and oral mucosa has been noted compared to the ACE-2 receptors
[36]. This could contribute to the high transmissibility and infectivity of SARS-CoV-2, particularly at viral entry points
[37][38].
Recent studies have reported a high degree of similarity in the NTD receptor-binding domain of different coronaviruses, as well as similarity in the structural alignment of the S1-NTD and GAL-3 of SARS-CoV-2
[35]. This indicates that both Neu5Ac and GAL-3 receptors may have similar -COOH terminal domains. The terminal galactose in the neutral sugar side chain of the polysaccharide enables MP to bind specifically to GAL-3 CRD
[39]. GAL-3 CRD has an affinity for β-galactosides and MP is a sugar molecule with an abundance of β-galactose
[40]. This enables MP to antagonize the GAL-3 β-galactoside protein by binding tightly to it and modulate its bioactivity
[41]. It is reasonable to assume that the β-1,4-galactan of the neutral sugar chain in MP has the potential affinity to bind specifically to the -COOH terminal of the Neu5Ac and/or GAL-3 receptors. This inhibits the attachment of the S1-NTD of SARS-CoV-2 to the host cell (
Figure 1). Although, a detailed investigation of the mechanisms involving GAL-3 binding and/or inhibition by MP will provide a means of testing this molecular hypothesis and identifying the particular pectin-derived components responsible for the effects.
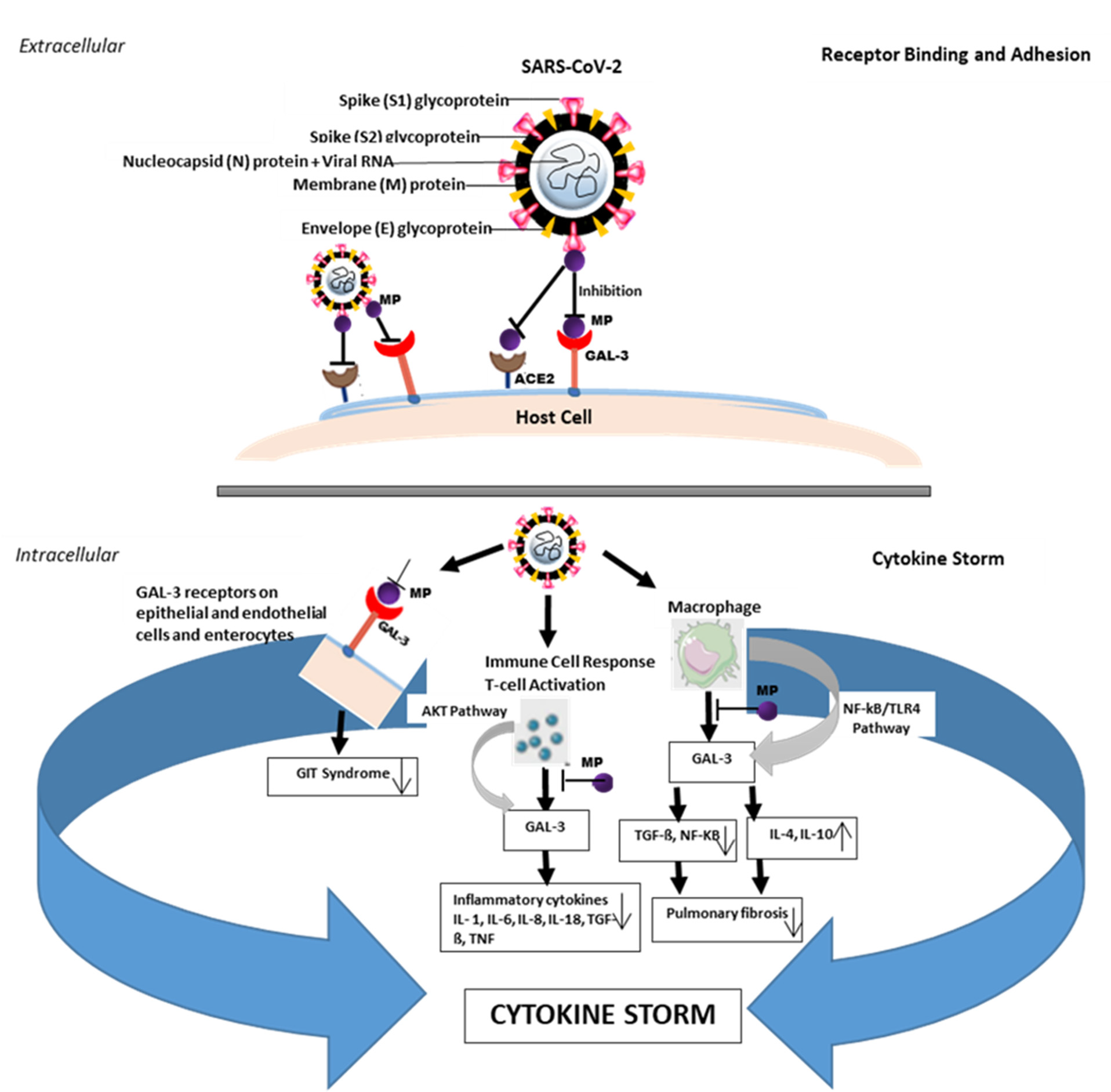
Figure 1. Effect of modified pectin (MP) associated with galectin-3 (GAL-3) during severe SARS-CoV-2 infection. Receptor binding and adhesion: MP binds to the GAL-3 receptor to inhibit SARS-CoV-2 attachment and adhesion to the host cell surface. During severe SARS-CoV-2 infection, the activation of T cell immune response through AKT and NF-KB/TLR4 pathways induces the release of GAL-3 and increase inflammatory cytokines such as interleukins (IL-1, IL-6, IL-8, IL-18) in circulating macrophages and monocytes, resulting in a feedback loop that may contribute to the development of the cytokine storm. This elevates the levels of TGF-ß and NF-KB, leading to pulmonary fibrosis. MP binds GAL-3 at the initiation and/or activation of the immune response stage, epithelial membranes, endothelial, and enterocytes to inhibit cytokine feedback and also prevents gastrointestinal tract (GIT) syndrome. GAL-3 inhibition by MP prevents or reduces the release and/or levels of the inflammatory cytokines which contributes to preventing pulmonary fibrosis.
The knowledge of antiviral compounds containing sugar or sugar analogs that may be used to prevent coronavirus from attaching to its sugar co-receptor
[31] has led to unraveling the antiviral potential of pectin and related flavonoids. A study has shown that plants can protect themselves against virus infection by silencing virus-induced genes stimulated by pectin methylesterase
[42]. This suggests that pectin methylesterase may contain compounds that mediate the prevention of viral infection in their host. A pectin polysaccharide from a plant named ‘
Portulaca oleracea’ containing galacturonic acid, galactose, and glucose with small amounts of arabinose and rhamnose showed significant inhibition against herpes simplex virus type 2 (HSV-2) with a selectivity index of more than 20
[43]. Similarly, pectin has high inhibitory effects of 179 μg/mL
−1 and 58 μg/mL
−1 against herpes simplex virus type 1 (HSV-1) and the poliovirus, respectively in Hep-2 cells
[44]. This further suggests that the interaction of pectin with the cation amino acids glycoprotein-binding site in HSV-1 and the anion sulfated/carboxyl group heparin-sulfate chains of the cell membrane impedes viral initiation and replication at the initial early stage
[44][45].
The S glycoprotein on the NTD is the major antigen on the viral surface that neutralizing antibodies can attack during an infection, hence preventing viral entry
[46][47]. It is pertinent to mention that the attachment of SARS-CoV-2 to the cell surface for replication through viral spike protein has multiple potential therapeutic targets. Although the relationship between GAL-3 and SARS-CoV-2 is also mixed, it is still unclear if it is pro- and/or anti-SARS-CoV-2. Furthermore, investigating the possibility of having similarities in the molecular structure of S1-NTD and MP could decipher the potential of a direct binding affinity of the sugar galactan to the CRD of SARS-CoV-2. This will provide an understanding of the interaction of N- and O-linked glycosylation sites of the spike glycoprotein with the carboxyl group on the MP galactan CRD in SARS-CoV-2 infection.
Some natural bioactive compounds and flavonoids such as hesperidin and hesperetin which are related to citrus pectin have demonstrated the potency of the antiviral effect. Hesperidin in citrus peel contains a very interesting molecule with potential antiviral activity against SARS-CoV-2
[48]. Studies reported that the inhibitory activities of hesperidin against SARS-CoV-2 are through its binding affinity to the protease domain, the ACE-2 receptor-binding domain, and the spike glycoprotein receptor-binding domain
[49][50][51]. Hesperetin binds to the receptor domain by inhibiting the attachment of SARS-CoV-2 to the ACE-2 receptor
[52]. Further study revealed that citrus hesperidin and hesperetin have the highest binding affinity to the SARS-CoV-2 receptors spike glycoprotein (S1-NTD), protease, and ACE-2 by showing the lowest dock scoring, which indicates a high inhibitory potential against the viral infection and replication
[49].
2.2. Modified Pectin and Galectin-3 Response to the Cytokine Storm Effect
According to studies, cytokine inhibition is one of the best therapeutic methods for COVID-19 depending on the stage of infection in the patient. It was further suggested that therapies aimed at reducing hyper inflammation and lung damage should be administered at the severe (pneumonia) stage
[53][54]. Based on the human immune response against SARS-CoV-2 infection, changes in T lymphocytes subsets (lymphopenia) accompanied by the cytokine storm syndrome contribute to the progression of the disease and poor prognosis. During this period, lymphopenia is commonly observed with an increase in IL-6 and other inflammatory cytokines (pneumonia phase). Acute lung injury, high initial viral titers, and macrophage/neutrophil build-up in the lungs are all symptoms of severe SARS-CoV-2 infection, as well as a high level of pro-inflammatory cytokines such as the interleukins IL-1, -6, -8, -18 and monocyte chemotactic protein-3 in the blood
[55].
Patients with severe COVID-19 have significantly higher levels of GAL-3, tumor necrosis factor (TNF), IL-1, and IL-6 than those with moderate disease
[56][57]. Furthermore, GAL-3 regulates and possibly causes a dysregulated pattern of these pro-inflammatory cytokine expressions during infection through the AKT signaling pathways
[58]. Consequently, the inhibition of GAL-3 greatly reduces the level of these cytokines, which suggests that it could be useful in lowering the inflammatory consequences of COVID-19
[56][59]. Secreted GAL-3 produced by macrophages during injury promotes the upregulation and elevated level of TGF-ß receptors, leading to pulmonary fibrosis (fibroblast activation), observed to be one of the major complications of SARS-CoV-2 infection
[60][61]. The inhibition of GAL-3 has been shown to reduce adenovirus-induced lung fibrosis
[62]. Thus, it is worthwhile to investigate MP as a potential treatment through its GAL-3 inhibition for pulmonary fibrotic-related diseases including severe cases in COVID-19.
The unique anti-inflammatory bioactivity of MP in humans being connected to the sugar β-galactose-inhibiting cell signaling protein, GAL-3, is responsible for tumor cell proliferation and metastasis
[24]. This stimulates or modulates intestinal homeostasis and also plays a role in immunological modulation
[63]. Although GAL-3 inhibits the inflammatory response of the intestinal system via the GALT, which modulates macrophage signaling recruitment
[64], it can as well bind to cell surface receptors to create a clustering effect
[65]. Consequently, a higher concentration of GAL-3 at this binding site activates T cells with a possible evasion of the immune surveillance system. The initiation of MP binding to GAL-3 in addition to the possible Neu5Ac interaction linked to the ganglioside domain on the epithelial cell surface may inhibit the extracellular matrix interactions.
The RNA of SARS-CoV-2 has been presented in the gastrointestinal tracts and stool samples of COVID-19 patients
[66][67][68]. This implies that SARS-CoV-2 can infiltrate enterocytes and serve as a virus reservoir
[68]. It is noteworthy that a rising number of SARS-CoV-2 patients have reported the possible indication of gastrointestinal symptoms including diarrhea (2.0–10.1%), nausea and vomiting (1.0–3.6%), and abdominal pain in COVID-19 patients
[69][70]. Most of these patients infected with SARS-CoV-2 have mild gastrointestinal symptoms and a good prognosis after the infection indicates that the immune function is a strong defense against this virus. The SARS-CoV-2 binds to the ACE-2 receptors which are highly expressed on alveolar cells of the lungs, upper esophagus, and stratified epithelial cells, as well as other cells such as absorptive enterocytes from the ileum and colon, myocardial cells, and kidney proximal tubule cells
[71]. This explains why, in some cases, COVID-19 patients not only experience respiratory problems but also disorders of the heart, kidneys, and digestive tract
[72].
3. Future Directions
Despite evidence from numerous studies that MP inhibits various steps in cell–cell interactions, fibrotic, renal injury, and cardiovascular diseases by interacting with GAL-3, the details of the underlying mechanisms are still largely unknown. Although pectin-derived galactan binds specifically to GAL-3, the precise structural characteristics responsible for the optimal binding to GAL-3 causing pro-inflammatory mechanisms associated with immune modulation remain unknown. As variants of SARS-CoV-2 are evolving, the virus may switch in binding sites to new receptors, from the firstly known ACE-2 to newly discovered ones as in the case of GAL-3. This implies that virus inhibitors at the attachment and entry stage are key to mitigating SARS-CoV-2 replication in the host cell; hence, the quick development of these inhibitors should suffice. Despite a few uncertainties, there is a good level of tolerance with regard to the safety and non-toxic acceptance of MP oral consumption, with evidence that dietary and citrus pectin polysaccharide RGI fragments have a positive effect and good prognosis on some viral infections in vitro. Its potential to benefit COVID-19 patients by regulating the immune response system is a possibility yet to be determined; hence, both in vitro and in vivo studies should be explored.