Figure 6. The mechanisms for the preparation of electrospun zein nanoribbons using unspinnable surfactant solution as a sheath fluid, and their applications in removing the lead ions from polluted water. Reprinted with permission from
[40].
The difference between the traditional single-fluid blending process and the multiple-fluid process mainly lie in how to guide the fluids in an organized manner. Shown in Figure 7a,b are digital photos about the modified coaxial methods for creating zein nanoribbons. The upper-right inset shows a core-shell droplet, in which the core spinnable zein solution consisting of 30 g zein in 100 mL of a 75%/25% (v/v) ethanol/water mixture was surrounded by a transparent and unspinnable sheath fluid composed of 0.5% (w/v) sodium lauryl sulfate in 75%/25% (v/v) ethanol/water. An enlarged image shown in Figure 7b clearly tells the typical three steps of electrospinning, i.e., the compound Taylor cone (the upper-left inset), the straight fluid jet, and the bending and whipping regions with enlarged loops in the instable region.
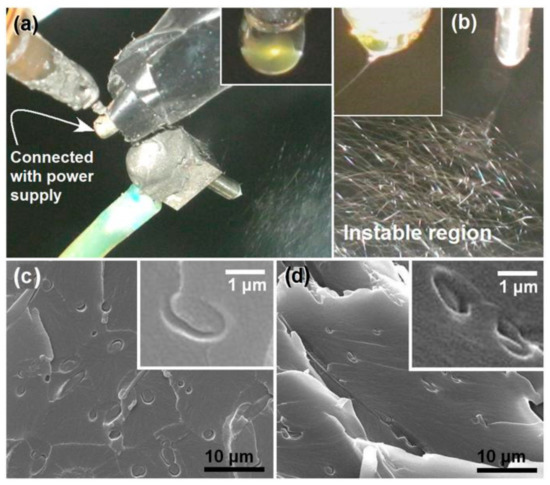
Figure 7. The working processes of modified coaxial electrospinning (
a,
b) using unspinnable surfactant solution as a sheath working fluid and the prepared zein nanoribbons (
c,
d). Reprinted with permission from
[40].
Cross-sections of different zein nanoribbons were prepared, which are compared in Figure 7c,d. The former image is zein ribbons prepared using the single-fluid electrospinning. The latter image is zein ribbons created using a modified coaxial electrospinning, in which unspinnable SDS ethanol aqueous solution was exploited as a sheath working fluid. By estimation, the surface area of zein ribbons from the coaxial process was enlarged 2.29 times than those from the single-fluid process, which was favorite for lead ion adsorption. By exploiting the favorable interactions between metal and protein, the zein nanoribbon mats were used to treat Pb2+-polluted water. Adsorption results indicated that the adsorption process can be described using the pseudo-second-order model. Isotherm data fitted well to the Langmuir isotherm model, with a maximum adsorption of 89.37 mg/g for the nanoribbons prepared from the modified coaxial process. Desorption results showed that the adsorption capacity can remain up to 82.3% even after 5 cycles of re-use. These positive results apparently demonstrated that the unspinnable working fluids utilized in the modified coaxial electrospinning were able to stabilize the preparation process, to further downsize the nanoproducts’ width, and to improve their functional performances in environmental remediation.
4.2. Pure solvent as a sheath fluid to create oligomer-loaded functional nanofibers for removing mercury ions
For the traditional single-fluid electrospinning, the filament-forming polymeric matrices must have enough molecular weight to support enough physical entanglements for forming electrospun fibers within their electrospinnable windows
[41]. Few reports can be found on the directly electrospun nanofibers of oligomers because they haven’t enough molecular weight and in turn no enough physical entanglements in their working fluids. These oligomers solutions are often un-electrospinnable and can’t be converted into nanofibers.
However, in a recent investigation, an oligomer Poly(2-aminothiazole) (PAT) was synthesized, and then this functional oligomer solution (0.1 g of PAT was dissolved in a mixed solvent of 2 mL DMAC and 2 mL ethanol) was mixed with a cellulose acetate (CA, 1.44 g of CA was dissolved in 8 mL of acetone) solution to create composite nanofibers. The sheath fluid was pure solvent acetone, which was pumped quantitatively through another booster to another inlet of a concentric spinneret for a smooth working process.
Figure 8 shows two representative high-magnification TEM images of the PAT/CA fibers, which clearly present their core-sheath nanostructures. The sheath thickness ranged from 35 to 205 nm, and the core thickness ranged from 105 to 480 nm. The sheath section was mainly composed of small PAT nanoparticles with only few nanoparticles appeared in the core section. This phenomenon is beyond anticipation because there was no any solutes in the sheath working fluid. The resultant core-shell structures should be a reason of solid phase separation during the modified coaxial processes. The concrete forming mechanism is still waited to be further disclosed.
Figure 8. Both (
a) and (
b) are representative TEM images of 0.1 g PAT/1.44 g CA nanofibers with unexpected core-shell nanostructures. Reprinted with permission from
[41].
The Hg(II) adsorption properties of the PAT/CA composite nanofiber mat from polluted water was tested. The adsorption capability was highly dependent on the pH value of solution, with a maximum at pH 6.5. The isotherm data fitted well to the Langmuir isothermal model. The adsorption kinetics data fitted well to the pseudo-second-order model. At a temperature of 298 K, the maximum adsorption capacity of 177 mg/g for PAT/CA fiber membrane with a very low PAT percentage (6.5 wt.%) was calculated. Through conversion, the adsorption capacity of the PAT in the composite fiber membrane is much larger than that of the nanoparticle-type PAT. The desorption experiments revealed that the desorption percentage remained at 80.2% after being used for three times. Here the unspinnable sheath solvent smoothed the electrospinning processes to prevent the spinneret clogging of CA, resulted in solid phase separation to lead to a surface distribution of functional ingredient, i.e., PAT particles, and thus provided an improved functional performance of removing Hg(II) from water. Certainly, the PAT-loaded nanofibers, as a non-woven mat, were more convenient than the PAT powders themselves for environmental remediations and less possibility of secondary pollutions due to nanoparticles themselves.
4.3. Nanocoating of inorganic materials on polymeric fibers for antibacterial applications using unspinnable salt solution as a sheath fluid
Through the modified coaxial electrospinning, it is possible to directly distribute the inorganic particles on the surface of polymeric nanofibers, in which nanosuspension can be explored as the sheath working fluid. Unfortunately, the inorganic nanoparticles are very easy to precipitate during the working processes, which may stop the continuous preparation. However, a combined strategy based on the modified coaxial electrospinning using salt solution as a sheath working fluid can overcome this issue.
With a solution of 15% (w/v) polyacrylonitrile (PAN) in N, N-dimethylacetamide (DMAc) as the electrospinnable core working fluid, a 10% (w/v) AgNO3 DMAc solution was employed as a sheath fluid, a modified coaxial process was successfully carried out to create the salt coating PAN nanofibers. The electrospun nanofibers were then exposed to 254 nm ultraviolet light for 24 h to reduce the Ag+ to Ag nanoparticles (NPs). The prepared products are shown in Figure 9a,b. Both nanofibers have kind linear morphology with the Ag NPs uniformly distributed on the PAN surface. This is very useful for the Ag NPs to exert their functional performances.
The influence of the key parameter, i.e., the sheath-to-core fluid flow rate ratio was investigated. The results demonstrated that the smaller flow rate of AgNO
3 solution of 0.1 was not able to follow the core PAN fluid jet during the fast bending and whipping process. This results in a separation of the sheath AgNO
3 solution and a non-uniform deposition of AgNO
3 on the surface of nanofibers (
Figure 9c,d). Thus, a higher sheath-to-core fluid rate ratio of 0.2 was selected to carry out the experiments, which resulted in a more homogeneous distribution of AgNO
3 on the surface of nanofibers. When the Ag
+ on the surface of PAN nanofibers was reduced to Ag NPs, the nanofibers from a larger sheath fluid flow rate ratio had a more uniform but smaller size, and a tighter distribution than those from a smaller ratio (
Figure 9c,e). It is anticipated that reduction of Ag
+ to Ag NP on the surface of nanofibers should be beneficial to long-term stability of the system and prevent Ag leaching from the fibers. These Ag NPs-loaded nanofibers showed fine antibacterial performances and should be candidates for friendly ecological environmental applications.
Figure 9.The Ag nanoparticles coating nanofibers (a,b) and the mechanisms for fabricating inorganic nanoparticles distributed PAN hybrids for antibacterial applications (c–e). Reprinted with permission from [42].
The popularity of electrospun nanofibers has a close relationship with their small diameters, huge surface area and large porosity [43][44][45]. However, for a single-fluid electrospinning process, the treated fluids must be electrospinnable to keep the formation of solid linear nanofibers, or they will be degraded into particles or even wet films [31][46]. The modified coaxial electrospinning, with an unspinnable sheath fluid on a spinnable core fluid, can’t be utilized to prepare some advanced nanostructures presently such as hollow fibers from a traditional coaxial processes [47][48], which can be viewed as a special core-shell structure with an empty core. However, all the liquids, containing or not the filament-forming polymers, can be converted as a thin film on the solid core fibers to take advantages of the nanofibers’ unique properties. This give a hint on many new possibilities in future. There are many publications about the environmental applications of electrospun composite nanofibers, in which the guest functional ingredients scattered within host polymer matrices [49][50][51][52]. With the valuable hints from these investigations, new types of nanofibers can be fabricated using the similar raw materials for an improved functional performance. The functional ingredients can be prepared as their owns’ working fluids in the sheath section to superficialize them on the solid hybrid nanofibers. Meanwhile, the pure polymers core may increase the mechanical properties of the core-sheath structures.
5. Conclusions and Perspectives
As the fast development of electrospinning, modified coaxial electrospinning is upgraded from the traditional one and shows strong vitality. Its usefulness lies in both stabilizing working process for creating high quality monolithic nanofibers, but also in generating solid core-shell nanostructure and nanocoating. What is more, almost any kinds of functional raw materials, regardless of filament-forming property, can be treated into nanofibers to exert their functional performances by taking advantages of the unique properties of electrospun non-woven mats. The above-mentioned several examples demonstrated that the modified coaxial processes can be utilized to manipulate the nano products’ shape and size facilely, to incorporate oligomer into the nanofibers, and to coat inorganic salt on the surface of polymeric nanofibers. These preparations are impossible using the traditional processes. Based on the combination of modified coaxial electrospinning and the tremendous active ingredients for treating pollutants in our environment, a new platform can be built to develop more and more functional nanostructures for environmental remediation.
The new nanomaterials and the corresponding applications resulted from the modified coaxial electrospinning using unspinnable liquid as a sheath working fluid is still at its infant time period. It is anticipated there are more and more possibilities in future, an unspinnable fluid can be treated with one or more spinnable fluids in many ways such as as tri-axial
[53][54] and side-by-side
[55]. Electrospinning and the electrospun nanofibers may find their applications in almost all the environmental issues such as gas pollution, water pollution and solid waste. They can also find applications for a better ecological environment, such as producing antibacterial materials, anti-UV materials, noise reduction and electromagnetic shielding
[56]. Similarly, modified coaxial electrospinning and the corresponding nanofibers and nanostructures can upgrade these applications and generate new materials from an even wide selections of raw materials such as inorganic NPs, carbon nanotube, graphene and small molecules. In traditional monolithic nanofibers, the active ingredients are homogeneously distributed all over the polymeric matrices, means that most of them are buried into the nanofibers and can’t play their roles for removing the pollutants
[57]. With the unspinnable solution as a sheath working fluid, a wide variety of functional ingredients (such as cyclodextrin) can be effectively distributed on the surface of polymeric nanofibers
[58][59], and thus their functional performances on environmental remediation can be easily enhanced. Certainly, the modified coaxial electrospinning can further expand its capability of creating novel functional material through a combination with other spinning methods or numerous traditional physical and chemical manners
[60][61].
Just as the traditional electrospinning, one of the most important challenges for the modified coaxial electrospinning is to create nanofibers on a large scale
[62][63][64]. Another important challenge is get more and more knowledge about the special working processes
[65], here the key point is about the unspinnable sheath fluid’s role during the transmission of solvents through air-fluid-fluid interfaces and the related solidification mechanism of electrospun nanostructures. Certainly, the structure-performance relationships for environmental and also other applications based on the nanoproducts prepared using modified coaxial electrospinning should be a very interesting topic deservin.