Drop-in biofuels have a plant based origin and can be blended gradually into the existing fuel mix. The usage of drop-in biofuels ensures that little to no adjustments are required relative to existing fuel infrastructure. Non drop-in biofuels include alcohols such as (m)ethanol and gaseous fuels which cannot be used in a diesel engine without adjustments. In this study, the potential of drop-in biofuels is addressed. Based on their suitability as a marine fuel and their drop-in character, five drop-in biofuels are selected. These fuels include Fatty Acid Methyl Esters (FAME), Hydrotreated Vegetable Oil (HVO), Fast-Pyrolysis with full hydrodeoxygenation (FP), Hydrothermal Liquefaction with full hydrodeoxygenation (HTL) and Gasification with consequent Fischer–Tropsch synthesis (GFT).
1. Introduction
The shipping sector is responsible for about 90% of international freight transport
[1]. Emitting 2.8% of worldwide Greenhouse Gas (GHG) emissions, the share in air pollution of the shipping sector is about the size of Germany
[1][2]. Although the relative emissions per unit of cargo are low compared to other transport modes, the total share in emissions of the shipping industry is significant
[3][4][5]. At this stage, the major part of the world shipping fleet still runs on fossil fuels. In addition, the harmful emissions caused by the combustion of fossil fuels, other factors such as the finite oil supply and the sustainability of the oil supply chain create the need for alternative propulsion solutions
[6].
In order to decrease air pollution caused by the maritime industry, the International Maritime Organization (IMO) implemented regulations that limit nitrogen oxide emissions and the use of high sulfur fuels. However, regulations that limit GHG emissions from shipping are at this stage non-existent. The European Union (EU) implemented the Renewable Energy Directive II (RED II), which contains an obligation for the share of renewable fuels in the transport sector, but shipping is excluded. Nevertheless, the IMO has set the ambitious goal to decrease absolute GHG emissions from shipping by 50% by 2050 compared to 2008 levels.
To reach this target, Bouman et al.
[7] evaluated several potential emission reduction measures for shipping. This analysis showed that biofuels have a considerable potential to reduce GHG emissions emitted by ships. Biofuels are one of the few renewable fuels that are commercially available at this stage. The first generation of biofuels was primarily based on plant oil but this could result in indirect land use and deforestation. This is a concern when striving for sustainable shipping
[8]. The second generation of biofuels does not have this issue, and the optional sources are outlined also in the mentioned RED II of the EU. Additionally, biofuels contain negligible amounts of sulfur and are in line with current regulations. A distinction can be made between drop-in biofuels and non drop-in biofuels. Biomass types used as feedstock for the fuels were categorized using the categorization shown in
Table 1.
Table 1. Feedstock categorization. Partly adapted from De Jong et al.
[9].
Drop-in biofuels have the potential to decrease GHG due to the principle of a short-carbon cycle. In contrast to fossil products, the biomass used as a feedstock for biofuel production absorbs CO
2 from the air during their lifetime. When the biofuel is combusted, the same amount of CO
2 is released into the air. When considering waste products for this, there is no competition for land use in that manner. The net emission are, therefore, considered zero. However, biomass is not evenly spread across the globe, which might cause regions to import or export large quantities of feedstock
[10][11]. Therefore, the production and transport emissions cannot be disregarded and should be considered as emissions for the fuel. Hence, production and transport largely determine the sustainability of the biofuel. This induces the need for both transport and production of biofuels. Therefore, to reduce costs and emissions, both production and transport need to be optimized.
2. Potential of Drop-in Biofuels for the Maritime Industry
2.1. Costs Versus Emissions
The multi-objective optimization induces a trade-off between costs and emissions. The resulting marginal costs of GHG reduction for all scenarios are visualized in Figure 1. In every run, this trade-off is reconsidered to reach the highest GHG reduction against the lowest costs. A clear trade-off between costs and emission reduction is visualized. The most beneficial trade-off is found to be around 80% GHG reduction compared to Heavy Fuel Oil (HFO), which corresponds to the average fuel costs of around 900–1050 EUR/ton of biofuel.
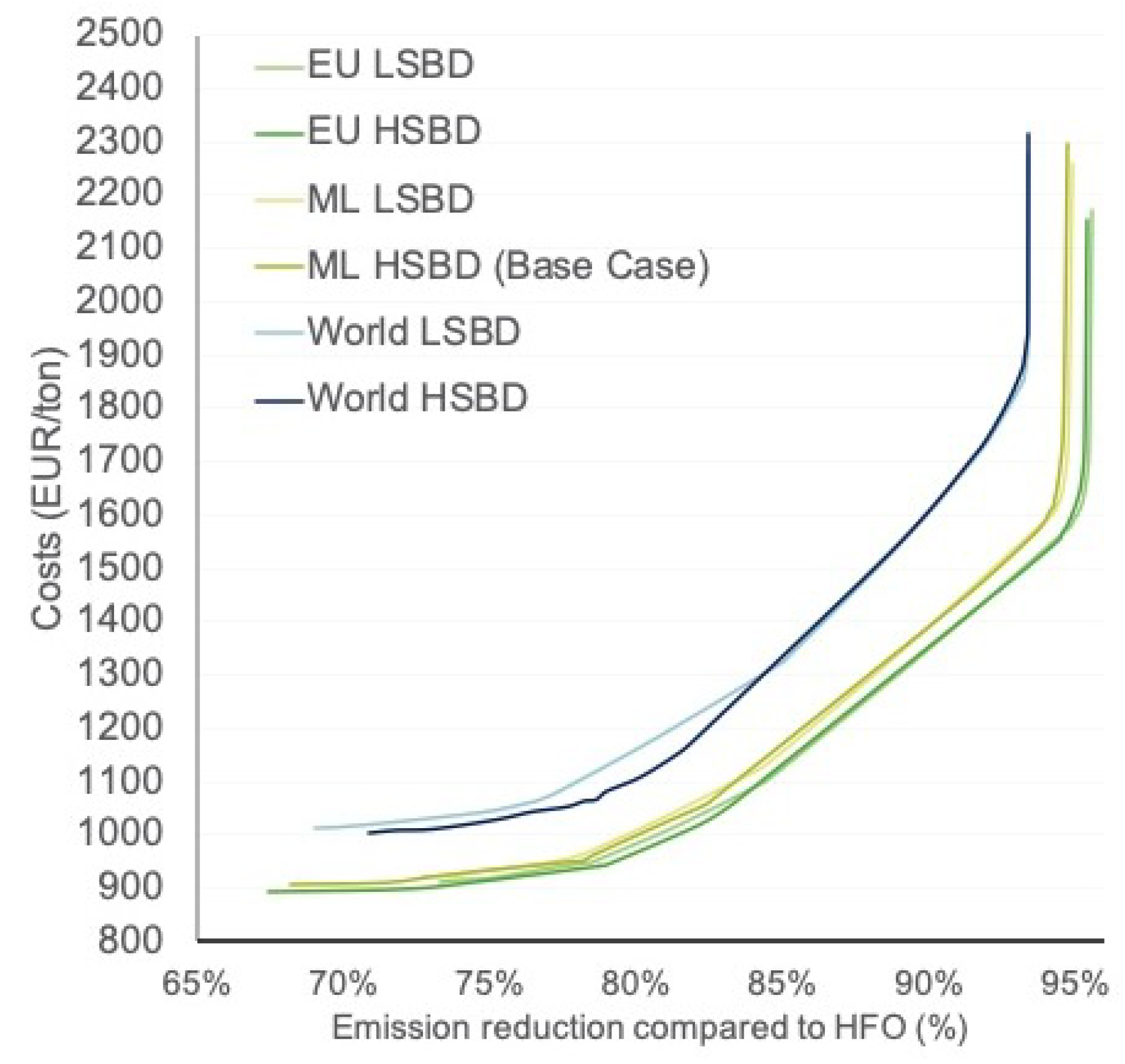
Figure 1. Marginal costs of Green House Gas reduction compared to HFO. A Well-to-Wake emission factor of 87.5 gCO
2
In Figure 2, the average fuel costs per time period are displayed. These costs are obtained by dividing the total expenses of that time period by the amount of fuel produced during that time period. It should be noted that these are average costs; in reality, different costs would be allocated to different fuel types. It stands out that, at first, fuel costs decline as a result of scale advantages and the use of cheaper feedstocks. However, after a strong decrease, costs gradually go up again. Rising demand induces the need for feedstocks that are increasingly difficult to collect, driving up the feedstock costs.
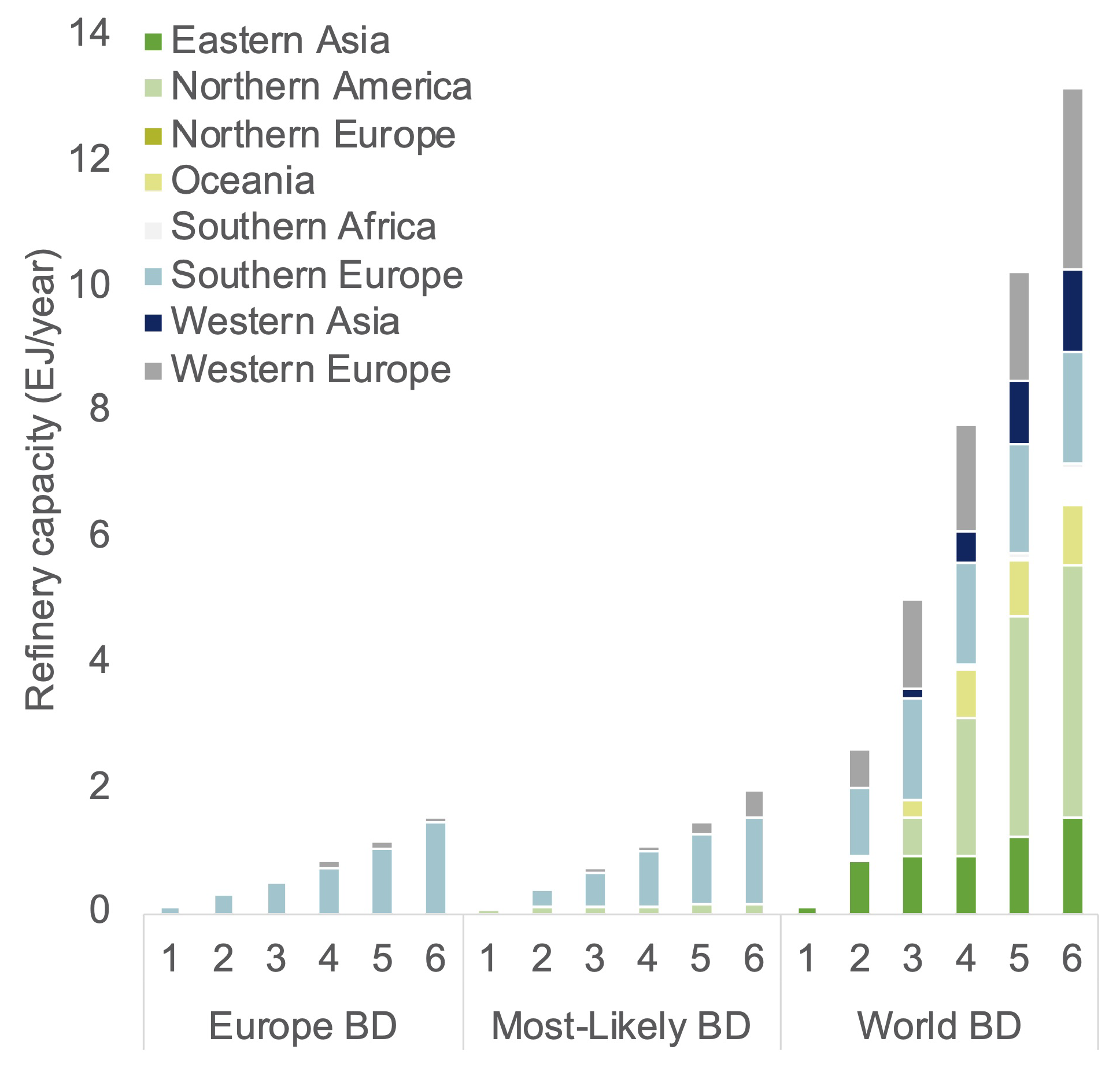
Figure 2. Projected average biofuel costs over the studied time period.
The results showed that around 20% of the costs are related to the raw materials, and 50% of the costs are associated with the production of the intermediate bio-energy carrier. The remaining 30% is related to the upgrading process. On the contrary, the upgrading process is responsible for around 85% of total GHG emissions. This is a result of the generous amounts of hydrogen required for the upgrading process. It is assumed that the used hydrogen originates from fossil sources. Using hydrogen from the of-gasses of the process or sourcing green hydrogen would make an enormous impact on the GHG reduction potential of drop-in biofuels. Additionally, it could be argued if drop-in biofuels for marine purposes require vast amounts of upgrading. Marine engines are often built for heavy-duty operations and residual or low quality fuel.
2.2. Technology and Feedstock Deployment
A total of five drop-in biofuel production pathways were considered in this study. In the first time period, only FAME and HVO were considered to be commercially available. After 2025, Fast-Pyrolysis (FP) and Gasification Fischer–Tropsch (GFT) were introduced. Hydrothermal Liquefaction (HTL) was introduced after 2030.
In all studied scenarios, HTL showed to be the most promising fuel both on economic and environmental performance. The main causes for this are the minor amount of upgrading required compared to Fast-Pyrolysis and the possibility in using a wide variety of feedstocks, including manure and sludges. However, the potential of HTL could be inhibited by its low TRL (Technology Readiness Level).
Feedstock deployment resulted in a mix of feedstocks, with a small preference for the use of agricultural residues. The reason for this mix can be partly attributed to the existing trade-off between costs, environmental performance and technological efforts
[12]. As an example, waste streams such as manure and Municipal Solid Waste (MSW) are cheap and have a high emission reduction potential. However, it is extremely difficult to obtain a constant stream that is suitable for the production of biofuel with steady quality. For residues from agricultural and forestry practices, feedstock costs rise when demand increases due to the need for feedstocks that become increasingly difficult to collect.
In Figure 3, the enormous refinery capacities required to reach the proposed targets are lined up for the three geographical demand scenarios. To place this in perspective, common biorefinery sizes mentioned in the literature are in the range of 1000–2000 dry ton input per day. This would correspond to an estimated biorefinery output of 0.0035–0.007 EJ/yr (using an average feedstock energy density of 18 MJ/kg and conversion yield of 0.6). In order to comply with the GHG reduction target of the IMO by 2050, this would impose a required investment in the range of 1100–2200 new biorefineries of that size worldwide during the studied time period. This provides an idea of the enormous challenges that lie ahead.
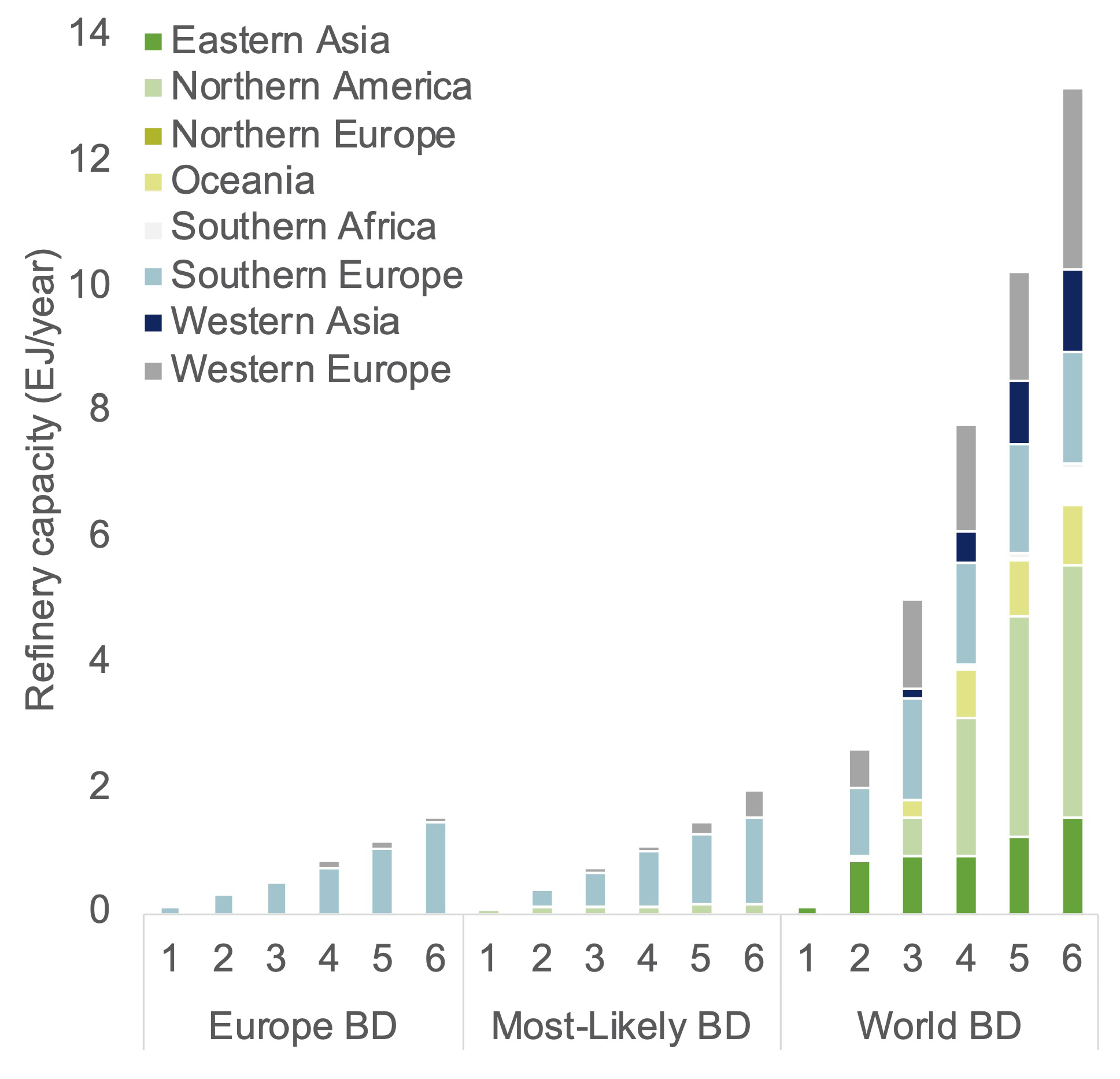
Figure 3. Required bio-refinery capacity per region to comply with the targets proposed in the developed scenarios. Capacity is given in the required refinery output. Integers represent the studied time intervals. 1: 2020–2025; 2: 2025–2030; 3: 2030–2035; 4: 2035–2040; 5: 2040–2045; 6: 2045–2050.
3. Conclusions
In order to measure the potential of drop-in biofuels for the maritime industry, a strategic supply chain optimization was performed to determine the economic and environmental performance of drop-in biofuels. Additionally, a comprehensive scenario analysis identified the availability of biomass and the required investments in new infrastructure and technologies to achieve the proposed GHG reduction targets of the RED II and IMO.
Concerning the economic performance of drop-in biofuels, the price gap with fossil products is still large. Although technological learning effects were not considered, the production costs of drop-in biofuel were projected to initially decrease. This is mainly caused by the gradually growing influence of economies of scale in combination with the introduction of new technologies that are able to use cheaper feedstocks. However, drop-in biofuels could also be the victim of their own success. In contrast to other renewable fuels, increasing demand results in the need for more expensive feedstocks that are difficult to collect.
The possible GHG reduction potential of drop-in biofuels was found to be between 68 and 95% compared to HFO for a cost of 850–2300 EUR/ton. Emission reductions of 80% compared to HFO are estimated to be achieved at fuel costs of around 900–1050 EUR/ton.
The availability of oils and fats is a severe barrier to the scalability of drop-in biofuels. Additionally, it could be argued if dependency on waste oils for the production of marine fuels is desirable. This increases the urgency for the rapid development of new conversion technologies that pave the road for the usage of more abundant feedstocks. It has been shown that in order to achieve the proposed emission reduction targets, enormous investments in new biorefineries are required. This entails investments in smaller processing facilities located near the feedstock source and larger refinery hubs located near ports.
In conclusion, drop-in biofuels offer a significant GHG reduction potential for the shipping industry. These fuels can be used without adaptations to existing fuel infrastructure. FAME and HVO are already commercially available, but limited feedstock availability induces the need for investments in new conversion technologies. When these technologies become available, enough biomass is domestically available to serve a large part of the shipping industry.