1. Introduction
Hydrogels can be defined as network structures configured at two or three dimensions which have the ability to imbibe high amounts of aqueous solutions or biological fluids
[1]. This affinity for water adsorption is mostly related to the presence of hydrophilic (e.g., amide, amino, carboxyl, and hydroxyl) groups on their molecular chains
[2]. Due to the presence of these groups, several physicochemical properties have contributed to the classification of hydrogel systems such as porosity, structure softness, swelling capacity, and elasticity
[3]. These properties are more similar to biological system than those of any other synthetic biomaterial
[4]. To reinforce their structure and strengthen their mechanical force, hydrogels are cross-linked either by using physical mechanisms or chemical cross-linkers
[5]. The cross-linkers in the polymer network are formulated by covalent bonds, hydrogen binding, van der Waals interactions, or by physical entanglements
[6]. Furthermore, the hydrogel system becomes more solid and insoluble in any solvent, once its polymer chains are cross-linked
[7]. Such hydrogels can be assembled with cryo-gelation
[8], gas foaming
[9], micro-emulsion formation
[10], freeze-drying
[11], and porogen leaching
[12]. It is reported that the porous capacity and swelling behavior of ahydrogel mostly depend uponcross-linkers
[13], chemical structure of the repeating unit or chemical composition
[14], network structure
[15], solvent concentration, surrounding medium, polymer molecular weight, quality of solvent, and the specific stimulation. Hydrophilic hydrogels show distinctive properties due to adsorption of high amounts of water in their structures compared to hydrophobic polymericnetworks. Hence, hydrophobic groups may collapse in the presence of water. The collapsed chains minimize their exposure to water molecules, ultimately resulting in lower swelling ratios
[16].
Efficient delivery systems have been developed based upon on the understanding of their interactions with the biological environment, target cell population, target cell surface receptors, changes in cell receptors that occur with progression of disease, mechanism and site of drug action, drug retention, multiple drug administration, molecular mechanisms, and pathology
[17]. For these reasons, many reports have referred to bio-adhesive polymers as promising materials that should be integrated into the moieties of nanoparticles
[18]. This is in part due to their reactive potential and good penetration into stomach and mucosal layers. Bio-adhesive polymers are classified according to their interactions with biological systems into subgroups: mucoadhesive polymers which can penetrate mucus layers (e.g., ocular, nasal, vaginal, and small intestinal); gastro-adhesive polymers which can adhere onto stomach lining; and stimuli responsive polymers which can react to biological stimulants (e.g. glucose, enzymes, pH, and inflammation) ()
[19]. In addition to tablets, patches, gels, ointments, and film formulations; mucoadhesive nanoparticles have emerged recently to improve the controlled delivery of drugs. For nasal, vaginal, ocular, and oral administration, many nanoparticles have been optimized to possess mucoadhesive properties
[20][21][22].
Figure 1. Classification of hydrogel systems according to their response to biological systems.
2. Structure of Mucus Layers
Mucosal layers mainly composed of viscoelastic fluid are secreted by glandular columnar epithelial cells and contain around 99% mucin and water, with trace amounts of lipids, other proteins, and muco-polysaccharides
[23]. They line the walls of various bodily cavities such as gastrointestinal and respiratory tracts, as well as vaginal and ocular surfaces. They consist of a connective tissue layer (the lamina propria) above that is an epithelial layer, the surface of which is made moist usually by the presence of a mucus layer. The epithelia may be composed of a single layer (e.g., in the bronchi, stomach, and small intestine) or be multilayered/stratified (e.g., in the esophagus, vagina and cornea). The former contain goblet cells that secrete mucus directly onto the epithelial surfaces and the latter contain (or are adjacent to tissues containing) specialized glands such as salivary glands that secrete mucus onto the epithelial surface. Mucus is a visco-elastic gel which contains mucin as the main dry weight component, typically at 2–5% (w/v), with smaller proportions of lipids and other proteins
[24].
Mucins consist of high molecular weight glycoproteins encoded for by more than 20 different MUC genes. Mucins are either secreted or membrane-bound, and their expression pattern varies with their physiological site. In the gastrointestinal tract the membrane-bound MUC1, MUC3, MUC4, MUC12, MUC13, MUC16, and MUC17 proteins are expressed in addition to the secreted MUC2, MUC5AC, MUC5B, MUC6, and MUC7
[25]. MUC2 and MUC5A are mucins secreted in the small intestine and the stomach, respectively. The protein structure of mucin is rich in repeats of serine, threonine, and proline. These domains are heavily glycosylated with fucose, galactose, sialicacids, N-acetylglucosamine (GlcNAc), and N-acetylgalactosamine (GalNAc)
[26]. The oligosaccharides account for 70–80% of the molecular weight of mucin and are believed to protect the protein core from degradation. The carbohydrate-bound ester sulphate residues and carboxyl groups of sialic acids provide mucus with a net negative charge
[27]. Thus, the charge of the attached oligosaccharides causes intestinal mucus to bear a more negative charge, as compared to gastric mucus. The glycosylated regions of mucins are hydrophilic, whereas the non-glycosylated protein domains are hydrophobic. These naked domains are rich in cysteine, and disulphide bonds link the mucin monomers. On average, mucins are composed of four monomers with each monomer having four to five glycosylated domains
[28]. Some of the lipids in mucus are covalently linked to the non-glycosylated domains of mucin, while most phospholipids adsorb and form a hydrophobic luminal lining of the mucus. The anatomic structures of mucosal layers found in different organs are shown in
[29].
Table 1. Comparison of mucus membranes in different organs.
Mucus Membrane
|
Mucus Surface Area
|
Thickness of Mucus Layer
|
Layers
|
Turnover Time
|
Buccal
|
30 cm2
|
0.1–0.7 mm
|
Epithelium, basement membrane, and connective tissues
|
5–6 days
|
Nasal
|
160 cm2
|
5–20 μm
|
Both keratinized and nonkeratinized epithelial cells
|
10–15 min
|
Ocular
|
|
3–10 μm
|
Pithelium, Bowman’s layer, stroma, Descemet’s membrane, and endothelium
|
15–20 h
|
Vaginal
|
6–10 cm2
|
10–15 layers
|
The epithelial layer consists of the lamina propia and stratified squamous epithelium
|
7 days
|
Rectal
|
300 cm2
|
50 µm
|
Single layer of cylindrical cells and goblet cells secreting mucus
|
90 h
|
6. Mucoadhesion and Its Relation with Certain Polymers
Several approaches have been designed to optimize hydrogel with bio-adhesive properties to overcome the issue of mucosal penetration and improve gastro-intestinal tract uptake
[37]. Such properties can be obtained by using marine mussels incorporating the catechol moiety 3,4-dihydroxy-L-phenylalanine (DOPA) in hydrogels to promote bio-adhesion
[38]. Bio-adhesion may also be improved by the interconnection of polymers having mucoadhesive properties such as chitosan, hyaluronic acid, polygalacturonic acid (pectin), alginate, and poly(acrylic acid) ()
[39].
Table 3. Polymers used to optimize mucoadhesive systems.
Polymer
|
Chemical Structure
|
Charge
|
Hydrogel Groups
|
Structure
|
Chitosan
|
Poly[β-(1,4)-2-amino-2-deoxy-D-glucopyranose]
|
Cationic
|
NH2 and OH
|
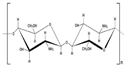
|
Hyaluronic acid
|
D-glucuronic acid and N-acetyl–D-glucosamine linked together through alternating β(1,3) and β(1,4) glycosidic bonds
|
Anionic
|
COOH and OH
|
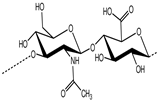
|
Alginic acid
|
β-(1,4)-D-mannuronic acid (M) and α-(1,4)-L-guluronic acid (G) units
|
Anionic
|
COOH and OH
|
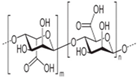
|
Polygalacturonic acid
|
Heterogeneous structure bonded via α (1,4) glycosidic linkage.
|
Anionic
|
COOH and OH
|
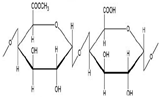
|
Carboxymethyl cellulose
|
Cellulose derivatives
|
Anionic
|
COOH and OH
|
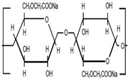
|
Poly(acrylic acid)
|
Polyacrylates
|
Anionic
|
COOH and OH
|

|
Additionally, lectin proteins canfurtherincrease the adherence of microparticles to the intestinal epithelium and enhance the penetration of drugs. Recently, lectins have been incorporated into nanoparticle moieties as a targetedtherapy that can bind to carbohydrate moieties attached to glycoproteins expressed on the surface of cancer cells
[40]. Thiolated polymers have also emerged as a promising approach that can be used for mucoadhesion and muco-penetration in drug delivery
[41].
The advantage of thiolated polymers is the ability of their free thiol groups to bind with cysteine domains in mucin
[42]. The inter- and intramolecular disulfide bonds that form between mucin and thiol groups, can lead to relatively improved stability, prolonged residence and disintegration time in the mucus, and more sustained drug release
[43]. Various thiolated polymers include chitosan–iminothiolane, poly(acrylic acid)–cysteine, poly(acrylic acid)–homocysteine, chitosan–thioglycolic acid, chitosan–thioethylamidine, alginate–cysteine, poly(methacrylic acid)–cysteine, and sodium carboxymethylcellulose–cysteine
[44].
It is demonstrated that chitosan adheres to mucosal surfaces within the body
[45] and canopen tight junctions between epithelial cells even through well organized epithelia
[46]. The electrostatic interaction between the positively charged amines on chitosan polymer chains (pKa = 6.5) and the negative sialic acid residues on the glycoproteins in mucosal fluid plays a role in the mucoadhesive properties of chitosan
[47]. Moreover, chitosan possesses NH
2 groups that can react with mucin forming electrostatic interpenetration
[48].
Polygalacturonic acid (PgA) is a natural polymer with a heterogeneous structure bonded via α (1,4) glycosidic linkage. Hanafy and his colleagues have demonstrated that PgA has limited dissolution in distilled water, Phosphate buffer saline (pH7.3), and acidic distilled water (pH3), resulting in white turbidity ()
[49]. This result is consistent with its hydrophobic properties
[50]. PgA is not degraded in the upper gastrointestinal tract due to its insolubility in acidic conditions
[51]. However, PgA can be dissolved in alkaline distilled water (pH 10), exhibiting a translucent yellow-green color. This means it can be hydrolyzed in the high pH environment of the colon. The cause of this dissolution is related to the pKa of its carboxyl group, i.e., at low pH it is protonated and the polymer is insoluble, while it is deprotonated at higher pH leading to polymer dissolution
[52]. It is reported that the combination of PgA with a second polymer into a composite may alter the degree of swelling and can change its mechanical properties
[53], improving both the stability of encapsulated drugs and the control of their release.
Figure 3. Dissolution of PgA by alkaline solution (figure adapted from
[49]).
It is reported that the addition of pectin to mucin in deionized water can form an aggregated compound, demonstrating an association between pectin and mucin
[54]. Despite both polymers bearing a negative charge, this reaction probably induces a hydrogen–molecular interaction, where electrostatic repulsion may cause the uncoiling of polymer chains, facilitating chain entanglement and bond formation
[55].
Poly(acrylic acid) (PAA) is a hydrophilic polymer
[56] that adopts a random coil conformation in solution. In their recent work, Hanafy and his colleagues have further demonstrated that these coils have swelling properties under certain ionic strengths and salt concentrations, leading to extended chain conformations in alkaline solutions ()
[49][57][58][59]. This is due to the COOH side chain which is pH responsive, i.e., protonated (COOH) at pH ≤ 5 while de-protonated (COO–) at pH > 5
[60]. In aqueous media of appropriate pH and ionic strength, the carboxylic groups ionize and develop fixed charges on the polymer network, generating electrostatic repulsive forces responsible for pH-dependent behavior, causing swelling or shrinking of the hydrogel structure
[61]. PAA is expected to form a hydrophilic corona. While in alkaline pH, the interaction of the swelling coils of PAA with water makes it more favorable for protection, as it adsorbs water at a weight many more times than that of its own.
[62][63]. Additionally, PAA is poorly soluble in water at low pH, causing structure shrinkage. This mechanical elasticity is predicted to protect the micelles during oral administration and through the gastrointestinal tract.
Figure 4. Poly(acrylic acid)extended at alkaline condition and shrunk at acidic condition(figure adapted from
[49] and
[56]).
Poly(acrylic acid) doped with cysteine can increase mucus glycoprotein adhesion via thiol–disulfide reaction. This reaction can significantly improve the mucoadhesive properties of PAA
[64].
Carboxymethyl cellulose (CMC) is a biodegradable polymer and a cellulose derivative. CMC is a good candidate for hydrogel formation because it has a good swelling capacity. Its carboxyl and hydroxyl groups are mainly responsible for hydrogel assembly due to their hydrogen intramolecular reaction
[65]. Hanafy and his colleagues fabricated a hydrogel system upon a surface of fluoro-magnetic nanoparticles by using polyethylene glycol and carboxymethyl cellulose. This hydrogel was constructed using layer-by-layer assembled polymers. Further, their hydrogel properties were used to derive activin-like kinase proteins
[66].
The adhesion of pectin
[51], polyacrylic acid
[60], hyaluronic acid
[67], and alginates to mucin makes them stronger electron donors than water. Also, hydroxyl groups can act as electron acceptors. Superior mucoadhesion is further demonstrated for polymers with low molecular weights because they can more easily interpenetrate the mucin network, or display intermolecular interactions with mucin molecules
[68][69][70][71].