1. Introduction
Metals, specifically heavy metals in effluent and sludge discharges from anthropogenic sources such as households, agriculture, manufacturing and process industries, are of major concern to environmental regulators
[1][2][3]. Notable amongst the metals and those that are classified as the most hazardous metal species are As, Cr, Ni, Cd, Pb, Co, Zn and Cu. Although the concentration of these metals very depending on the source, they are toxic and non-biodegradable, even at very low concentrations. Due to the high solubility of these metals, they are readily passed-on, absorbed and accumulated into the human body through the food chain, thereby causing cancers, neurological disorders, skin diseases, respiratory problems, congenital disorders, fertility decreases and chronic kidney damage
[4][5][6].
Knowing the aforementioned impact on water, soil and air, public concerns have increased over the years resulting in stricter legislations, most especially in more developed countries
[7]. However, various management and control schemes to address the adverse effects at their point sources and non-point sources have not achieved the extent of impact. While the presence of the metals in the discharges have been viewed as toxic and require complete removal, new age engineering considers them as a representation of a significant loss in raw materials. Sustainable treatment options in addressing the latter view, therefore, look at removal, recovery and reuse technologies (3Rs-Tech).
Ion exchange for the removal, recovery and reuse of metals is a widely known and effective treatment process. It is a selective, reversible and stoichiometric method that involves the displacement of ionic species by another ionic species in the exchanger
[8]. The exchangers serve as sorbents and are either resins or membranes. Although the mention of ion exchange usually refers to resins, ion exchange membranes (IEMs) have gained prominence due to their dimensional stability over resins
[9]. Wide spread use of IEMs include sea water desalination, water softening and purification, the chlor-alkali process, energy production and energy storage
[10][11][12].
The Donnan membrane process (DMP), commonly referred to as Donnan Dialysis, is an emerging green treatment process that integrates IEMs. The first usage of DMP is attributed to Prakash and SenGupta
[13]. The DMP involves the stoichiometric counter transport of ions across an IEM. As a concentration gradient driven process, DMP can be classified as a 3R-tech used in the recovery, separation and concentration of ions of interest from diluted solutions.
The DMP has often been interchanged with Diffusion Dialysis (DD) due to their indistinguishable principles of operation and application advantages. Whilst DD is utilized in the recovery of mineral acids or alkalis from waste acid and alkaline solutions, DMP is applied in the recovery of toxic or valuable heavy metal ions
[14][15][16]. The simple and easy to operate DMP system exhibits functional advantages over the conventional ion exchange process, electrodialysis (ED), chemical precipitation and pressure driven membrane processes. The DMP is an energy efficient, low installation and operational cost, non-risen regeneration and a non-fouling process that possess rural application benefits
[17][18][19][20][21]. Ion transport in a DMP occurs as long as the donor phase volume is greater than the receiver phase. expounds on the advantages and disadvantages of some metal removal processes.
Table 1. Advantages and disadvantages of selected metal removal technologies.
Process
|
Advantages
|
Disadvantage
|
References
|
Conventional Ion exchange
|
Low cost, high selectivity, little or no use of organic solvents, regeneration capability
|
Resin regeneration requires chemical addition, poor quality products, long production cycle, finding suitable resin is a challenge, process is highly pH sensitive.
|
[9][22]
|
Pressure driven membranes
|
Wide range application, simple configuration, high removal and rejection.
|
Susceptible to fouling, complex reverse cleaning process, additional pretreatment process is costly, internal and external concentration polarization depending on membrane process, expensive and non-recyclable drawing solutions for forward osmosis process, enrichment of contaminant in retentates causing secondary pollution, non-rejection of monovalent ions for nanofiltration, high energy demand for pressure pumps used.
|
[23][24][25][26][27][28]
|
Adsorption
|
Simple technology, wide range of metals selectivity, low cost local, materials readily available as natural absorbents,
|
High cost of absorbent, residue generation and disposal challenges, adsorbent regeneration complex and expensive, pH of solution affects sorption to binding sites, removal efficiency depends on type of sorbent, synthetic absorbent expensive to produce.
|
[29][30][31][32]
|
Chemical precipitation
|
Simple, low cost of precipitant, non-selective, shorter removal time.
|
pH adjustment is critical as precipitates can resolubilize, high residue generation and disposal, high chemical demand, large tanks at high installation costs, energy inputs required, generation of H2S for sulfide reagent, CO2 for carbonate reagent.
|
[33][34][35][36]
|
Bioremediation
|
Moderate cost, no waste generation, minimum or no disturbance to the soil, no ecosystem disruption, minimal energy requirement, large contaminants handled at a time.
|
Not recommended for non-biodegradable compounds, products after biodegradation can be more toxic, problematic upgrading from laboratory scale, contaminant migration through environmental resources, time consuming process, remobilization of stabilized contaminants due to changes in hydrological and geochemical conditions, inadequate benchmark values for field application, requires deep understanding of microbial process.
|
[37][38][39][40][41]
|
ED/reverse ED
|
Ion transport is rapid, effective in wide pH ranges, no phase change, not affected by osmotic pressure.
|
Stack clogging and membrane fouling, high energy consumption, skilled labor, compatibility of membrane and stacks materials to feed stream solution is highly required, current density limit, requires post treatment and pretreatment.
|
[42][43][44][45][46]
|
The DMP set-up consists of three phases, namely, the donor phase, which contains the ion of interest for recovery, the sweep phase, which contains the donating ion to enable the counter transport and, most importantly, the IEM, which controls and allows selective transport of the ions. Cation exchange membranes (CEMs) are used for removing, recovering, separating and concentrating metal ions. Anion exchange membranes are applied during specific treatment of harmful anions such Cl
−, F
−, HCO
3−, NO
3−, SO
42− and AsO
43− [47].
2. Ion Exchange Membranes (IEM)
Monopolar, amphoteric, bipolar and mosaic are the four (4) types of IEMs based on their charge functional groups and fixed ionic group pattern. Most IEMs for commercial applications are identified as monopolar with a single-line pattern
[48][49][50]. is a schematic diagram for the classification of IEMs.
Figure 1. Categorized ion exchange membranes. (
a) Positive or Negatively charged monopolar IEM, (
b) Amphoteric IEM, (
c) Bipolar IEM and (
d) Mosaic IEM adapted from
[49][50].
Depending on the charge group interconnection on the matrix phase of the membrane structure, IEMs are identified as homogenous and heterogeneous with varying properties and process advantages. In a homogeneous membrane, charged groups are bonded to a polymer backbone, while in a heterogeneous membrane, the ion exchange material is mixed with the polymeric matrix without chemical bonds between them
[51][52][53][54].
Homogeneous IEMs have higher conductivity, perm selectivity and a more balanced distribution of functional sites, but they are more costly to produce and have more complex manufacturing phases. Comparatively, heterogeneous IEMs have better chemical stability and mechanical properties over the homogenous ones
[55][56]. However, the low electrochemical properties of the heterogeneous IEMs are associated with ionic mobilization pathways, leakage of co-ions in the solution phase and the availability of inert fractions
[52].
IEMs are designed and produced to have desirable characteristics such as high permselectivity, high conductivity, good mechanical strength, structural stability and high chemical and thermal stability
[57][58][59]. The characteristics are also dependent on factors such as size of the ion exchange resin, resin loading, resin distribution, polymer used, solvent and method. Cation exchange membranes (CEMs) have proven higher stability in strong alkaline solutions than Anion exchange membranes (AEMs). Until recently, most commercially available CEMs and AEMs were homogeneous; Aciplex, Selemion Femion, Nafion, Fumasep, FKS, Ralex and Neosepta are known IEMs
[51][60][61]. illustrates a typical transport pathway of ions through a homogenous CEM (a) and heterogeneous AEM (b).
Figure 2. Ions pathway through a homogeneous CEM (a) and heterogeneous AEM (b).
Non-commercial membranes are often developed for performance evaluation and comparison with commercial membranes. These membranes are either synthesized or result from structural modification of existing membranes. To develop the surface, permselectivity efficiency and ion exchange capacity (IEC) of any membrane, various preparation and modification techniques are applied, which include phase inversion, irradiation and film etching, microfabrication, film stretching, sintering of powders, track-etching, electro-deposition, sol-gel process and coating (dip coating, in situ polymerization, plasma polymerization, interfacial polymerization)
[62][63]. However, surface engineering and modification is focused on the use of solvent-free technologies.
In short, IEM characteristics such as ion conductivity, hydrophilicity and hydrophobicity, ionic properties, embedded ion exchange groups, charge density and membrane-ion-affinity are the foundation for application in various ion exchange processes, which includes DMP
[50][64][65]. The selectivity transport functionality of the membrane (characterized by morphology and microstructural variation) for target ions in the midst of multivalent ions influences their choice to achieve various DMP separation objectives. For target metal ions, the CEM (a) is used.
The activation of CEMs prior to usage in a DMP system is essential to achieve a high membrane hydration. It ensures the setting up of transport pathways for the permeation of ions. Crucial to the conditioning process is the removal of impurities and factory defects from the surface of the membrane. Immersion and conditioning in acid is commonly adopted by researchers
[66].
The sequence of conditions commence with immersion in H
2O
2, rinsing in distilled water or boiling water and is proceeded with acid conditioning with HCl, H
2SO
4 and/or HNO
3 at an elevated temperature of ≤90 °C
[66][67][68]. The treatment chain is then completed by final rinsing in either deionized water at high or normal temperature. However, most treatments do not opt for HNO
3 conditioning. Further treatment of the CEMs with 1% dilute HCl for 3 hrs enhances ionic transport by increasing the inter-pore hydration of the membrane. Other procedures use NaOH neutralization in between two acid conditioning steps that alternate between HCl and H
2SO
4 at different treatment times and temperatures, including room temperature, for the same membrane
[69][70].
3. Donnan Membrane Cell
Four modules, notably the plate and frame, spiral wound, hollow fiber and the tubular type
[71][72][73], are known in the membrane industry. However, two modules are applicable in the DMP system as there is the requirement of separate solutions flowing on either side of the membrane for counter exchange of the ions. These are the plate and frame and the tubular modules. The plate and frame modules are one of the earliest in the membrane industry and consist of a flat sheet membrane and a mesh spacer sandwiched between two blocks and plates. The tubular module consists of smaller tubular compartment housing membranes that are fitted into a larger tube
[74]. Flat sheet modules have low performance characteristics, while tubular modules have medium performance characteristics, based on performance parameters such as promoting high cross flow rate, high filtering area to volume packing ratio and a pre-treatment requirement.
Various compartments to contain the donor and sweep phase solutions and membrane have been developed over the years. These modules are designed to meet main design criteria cited by
[17] for DMP. These compartments are mostly made from materials such as borosilicate glass, Plexiglas (C
5O
2H
8)
n, PVC (C
2H
3Cl)
n and Teflon (C
2F
4)
n. A simple two-compartment cell has seen development with the attachment of external donor and sweep side vessels. Flow patterns are set-up with compressed air (a), magnetic stirrer (b) and shaking blocks or baffles (c). In addition, turbulence at the membrane solution surface can be caused by increasing the flow rate of the electrolytic solution for a DMP compartment in d. Zhao et al.
[75] used a similar set-up as demonstrated in c and called it a point of use dialyzer. Additionally, cell arrangements vary and hybrid structures have included a 20 cell pair mounted with CEMs, 11 cells consisting of 5 feed and 6 sweep cells and a 3–4 membrane cell
[76][77][78].
Figure 3. Donnan Membrane Process Cell Designs: (a) a simple compartment with compressed air agitation; (b) Compartment with external vessels and a mixing unit; (c) Point of Use systems; (d) Donnan membrane rig.
4. Trends for Target Metal Ion
The Donnan membrane process applications cover various industries spanning from the mineral process to the water and wastewater treatment industry. Depending on the DMP configuration, operating variables that affect recovery, separation and concentration of target metal ions are the concentration and flowrates of donor and sweep phases, electrolytic sweep solution, valence of counter ion, pH, experimental duration, membrane type and morphology
[79].
Most researchers use the one-factor at a time (OFAT) approach to evaluate the transport of metal ions. In OFAT, one factor is varied while the other variables are kept constant. Using OFAT, multiple experiments cannot be run, while a high number of experiments makes it cost intensive and time and resource consuming, with the inability to assess the interactive effect of variable optimal settings
[80][81][82].
The statistical approach, also known as design of experiment (DOE), allows researchers to evaluate the independent and interacting effect of various process variables under consideration. Therefore, statistical models were developed that aid in process optimization
[83][84][85].
Two relevant polynomial models are often involved. The first model, as seen in Equation (3), is for special cases, and this includes first-degree models (d = 1). The second degree model (d = 2) is also expressed in Equation (4)
[86] as:
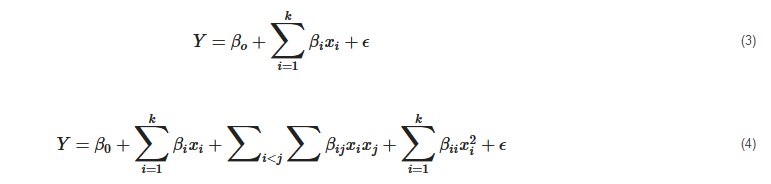
where Y, β0, βi, xi and ϵ are the characteristic response, constant term, coefficient, independent process variable and random experimental error at a zero mean, respectively.
A statistical approach has been used in only a few DMP studies involving target metal ions to assess the impact of process variables on recovery. A face centered central composite model developed for Al
3+ considered the donor phase concentration, donor phase flowrate, sweep concentration and sweep flowrate
[87]. Furthermore, screening studies for the four factors indicated that the sweep concentration had an insignificant effect on aluminum recovery
[88]. As such, a Box–Behnkein model was developed using the donor concentration, donor flowrate and sweep concentration as factors for the design matrix.
Kinetic models have been developed for monovalent (Na
+, K
+, Cs
+ and Ag
+), divalent (Ca
2+, Ba
2+, Mg
2+ and Sr
2+) and trivalent (Al
3+) metal ions based on Fick’s and Nernst–Planck’s equations for ion fluxes
[89][90][91][92][93][94]. Interestingly, all kinetic models for the mass transfer of the metal ions through the membrane have been conducted using the Nafion 117 membrane. Different commercially available Nafion membranes for possible DMP studies and their respective properties are presented in
[95][96][97][98][99][100][101][102].
Table 2. Commercially available Nafion membranes with their respective properties.
Nafion
|
Formation
|
Equivalent Weight (g eq−1)
|
Nominal Thickness (µm)
|
Basic Weight (g m−2)
|
N 115
|
Extrusion
|
1100
|
127
|
250
|
N 117
|
1100
|
178–183
|
360
|
N 1035
|
1000
|
89
|
175
|
NR 212
|
Solution casted
|
1100
|
50–51
|
100
|
NR 211
|
1100
|
25.4
|
50
|
XL
|
Reinforced
|
1100
|
27.5
|
55
|
HP
|
-
|
20
|
43.5
|
424
|
1100
|
180
|
540
|
1110
|
Extrusion
|
1100
|
254
|
500
|