Skin wounds can normally heal without problem; however, some diseases or extensive damage may delay or prevent healing. Non-healing wounds represent a serious and life-threatening scenario that may require advanced therapeutic strategies. The main task of skin stem cells (SSCs) is to replace, restore, and regenerate the epidermal cells that may have been lost, damaged, or have become pathologically dysfunctional.
1. Introduction
The skin is composed of two main layers, i.e., the epidermis and the dermis. Previously, another layer had been described within the skin, i.e., hypodermis
[1]; however, there is an ongoing controversy in this regard and the hypodermis is now considered as part of the dermis. The skin contains accessories, such as hair, nails, and sweat, and sebaceous glands
[2]. In addition, the skin is also populated by nerve receptors that can be triggered by external stimuli (e.g., touch, heat, pain, and pressure)
[3]. The skin layers have different thickness according to their anatomical location; for example, the epidermis can be very thin in the eyelids (0.1 mm) whereas it can be thicker in the palms and soles of the feet (1.5 mm). In contrast, the dermis can be ~30–40 times thicker in the dorsal area than the corresponding epidermal layer
[4].
The epidermis can be further sub-divided into strata with a unique cell composition, i.e., keratinocytes, dendritic cells, melanocytes, Merkel’s cells, and Langerhans’ cells. These epidermal layers are known as stratum germinativum, stratum spinosum, stratum granulosum, stratum lucidum, and stratum corneum. The first of these strata, also known as the basal cell layer, conforms the inner-most part of the epidermis
[4][5]. It is in this layer that different populations of stem cells (SCs) are located, and which, through extensive proliferation and differentiation, provide the great regeneration capacity of the skin and enable the generation of auxiliary structures, e.g., nails and sweat glands
[6]. It must be mentioned that the basal cell layer is not the only stem cell niche within the skin as these cells can also be found within the hair follicle (HF), interfollicular epidermis (IFE), and sebaceous glands
[6], all of which are contained within the basal layer itself. The stem cells within the skin are usually named after the niche in which they reside in, i.e., hair follicle stem cells (HFSCs), melanocyte stem cells (MeSCs), interfollicular epidermis stem cells (IFESCs), and dermal stem cells (DSCs). Regardless of their niche, these cells are collectively known as skin stem cells (SSCs) (
Figure 1).
Figure 1. General structure of the skin. The skin is composed of two primary layers: the epidermis and the dermis. The epidermis, hair follicles, and the dermis are the primary skin stem cells reservoirs. Among the different populations of stem cells are hair follicle stem cells, interfollicular epidermis stem cells, sebaceous gland stem cells, melanocyte stem cells, and dermal stem cells. * The dermis represents a larger adult stem cell reservoir than the hair follicle and epidermis put together. Three representative stem cell subpopulations from the dermis (dermal stem cells) including neural crest stem cells, mesenchymal stem cells-like dermal stem cells, and dermal hematopoietic cells are represented in the figure. Some elements of this figure were taken from the Mind the Graph platform, available at
www.mindthegraph.com (accessed on 27 September 2021).
The main task of these SSCs is to replace, restore, and regenerate the epidermal cells that may have been lost, damaged, or have become pathologically dysfunctional
[7][8]. For such end, a carefully orchestrated cell division, both symmetrical and asymmetrical, is required to both maintain the stem cell pool and produce lineage-committed cell precursors
[9]. Initially, SSCs were thought to be age-resistant, mostly because their number does not seem to dwindle through time
[10][11]. However, despite their longevity, SSCs eventually become unstable or dysfunctional and display a lower differentiation and self-renewal capacity
[12].
2. Skin Stem Cells and Wound Healing
2.1. Cell Signaling Pathways and SSCs
The health and maintenance of the skin is tightly regulated through the secretion of diverse cytokines, chemokines, growth factors, and the activation of specific signaling pathways
[13][14]. Mitogens such as insulin-like growth factor (IGFs), fibroblast growth factor 7 (FGF-7), FGF-10, and the epidermal growth factor receptor (EGFR) facilitate epidermal regeneration
[15]. Several signaling pathways have been linked to the development and regeneration of the skin; however, none of them seem as relevant or essential as Wnt, which regulates cell proliferation, differentiation, migration, and polarity
[16][17]. Somewhat unique—the Wnt signaling pathway can be activated through diverse means, one of which is known as the canonical Wnt/β-catenin pathway. This canonical pathway is typically active during tissue morphogenesis, thus regulating its development
[18][19]. Interestingly, Wnt signaling drives skin development and maintenance through both canonical and non-canonical signaling cascades
[18][19]. So far, 19 Wnt genes have been identified in the human genome
[18]. These proteins are secreted into the extracellular environment and bind to the frizzled (Fz) family of receptors (e.g., lipoprotein receptor-related proteins 5 and 6 [LRP-5/6], receptor tyrosine kinase like orphan receptor 2 [ROR2], and receptor like tyrosine kinase [RYK]) to activate various signaling pathways
[20][21]. These Wnt receptors can be blocked by different proteins, e.g., Dickkopf protein (Dkk), secreted frizzled-related protein (SFRP), or Wnt inhibitory factor (WIF), which regulate the activation of the signaling cascade
[22][23]. On the other hand, Wnt signaling can also be modulated by R-spondin and leucine-rich repeat-containing G-protein coupled receptor proteins
[24][25].
The HF, as a major stem cell niche within the skin, has been strongly associated with Wnt signaling during its induction
[26]. HF morphogenesis occurs in three main steps: the formation of the hair placode, HF organogenesis, and cell differentiation
[27]. These events are tightly regulated by Wnt, thus reaffirming its role as a master regulator. As a first step, dermal fibroblasts, stimulated by Wnt, induce the aggregation of epidermal basal cells, thus forming hair placodes. Thereafter, this placode express Wnt ligands that induce the formation of a dermal condensate
[28]. At the same time, the placode keeps growing and produces an invagination into the dermis that joins with the dermal condensate to form the primary hair germ (HG), the first structure of HF organogenesis. The epidermal cells penetrating the forming dermis generate the hair peg while the dermal condensate transforms into a dermal papilla (DP). The bottom section of the hair peg becomes thickened and forms the hair bulb, which encloses the DP. The differentiated epidermal cells within the hair peg will create the hair shaft. Cell differentiation starts once the developing HF reaches the subcutis. At this stage, the DP becomes thinner and completely enclosed, whereas the sebaceous gland starts its development in the upper HF. After this, a hair shaft raises from the skin surface and the HF reaches its maximal length
[29]. As previously mentioned, SSCs occupy multiple niches within the skin, including the basal layer of the interfollicular epidermis (IFE) and the bulge region of the HF
[30]. Consistently, Wnt also plays an essential role in the maintenance, activation, and differentiation of the SSCs located in these niches.
HF stem cells are split into two sub-populations, i.e., one residing in the bulge (hair follicle stem cells, HFSCs) and another localized in the secondary hair germ (sHG)
[31]. Bulge HFSCs remain mostly quiescent; on the other hand, sHG stem cells are active during HF formation
[32]. In contrast, the epidermis is continuously renewed by the basal cells in the IFE, whose pool remains constant thanks to their capacity for asymmetric division
[33]. In this regard, the hierarchical and stochastic models suggest how the cells in the basal layer of the IFE can be replenished
[34]. The former states the existence of slow-cycling SSCs within the proliferative units of the IFE, thus generating short-lived transit-amplifying cells (TACs) that later become differentiated. The stochastic model, on the other hand, proposes that the progenitor cells in the basal layer of the IFE have the same potential to create progenitors or differentiate into supra-basal cells
[35]. Due to their proliferative and multipotential capacity, SSCs are essential for skin regeneration and wound healing
[36]. Within the IFE, SSC populations can be classified as label-retaining cells (LRCs) and non-LRCs during their phenotyping. It must be mentioned that these subpopulations differ at the molecular level and commit into different lineages; regardless, they are functionally similar and are capable of replenishing the opposing niche upon injury
[37]. Interestingly, the maintenance of the IFE does not require a clear SSC or TAC hierarchy
[38].
2.2. Principles of Wound Healing and Skin Regeneration
The skin is an essential component in the protection against environmental hazards, e.g., UV light, pathogenic agents, and dehydration. However, the continuous exposure to such threats also compromises its integrity. Therefore, wound healing and skin regeneration are indispensable for the health and survival of higher organisms. Wound healing is a highly conserved mechanism among species, and includes processes such as inflammation, blood clotting, cellular proliferation, and extracellular matrix (ECM) remodeling
[39][40]. During the inflammatory phase, the wound becomes sealed by fibrin, which forms a temporary matrix occupied by immune cells whose task is to remove dead tissue and control infection. Fibroblasts are recruited afterwards into the site of the injury and secrete collagen, form granulation tissue, and promote angiogenesis and the recruitment of fibroblast-derived myofibroblasts, which contract the wound area. SSCs are mobilized into the site of injury at this stage to begin the process of re-epithelialization starting from the edge of the wound. Finally, new ECM components are secreted by both fibroblasts and epidermal keratinocytes, which also remodel the matrix through the expression of matrix metalloproteinases (MMPs). Amazingly, the regenerated skin tissue is able to regain ~80% of its normal strength in as little as 3 to 4 months (
Figure 2).
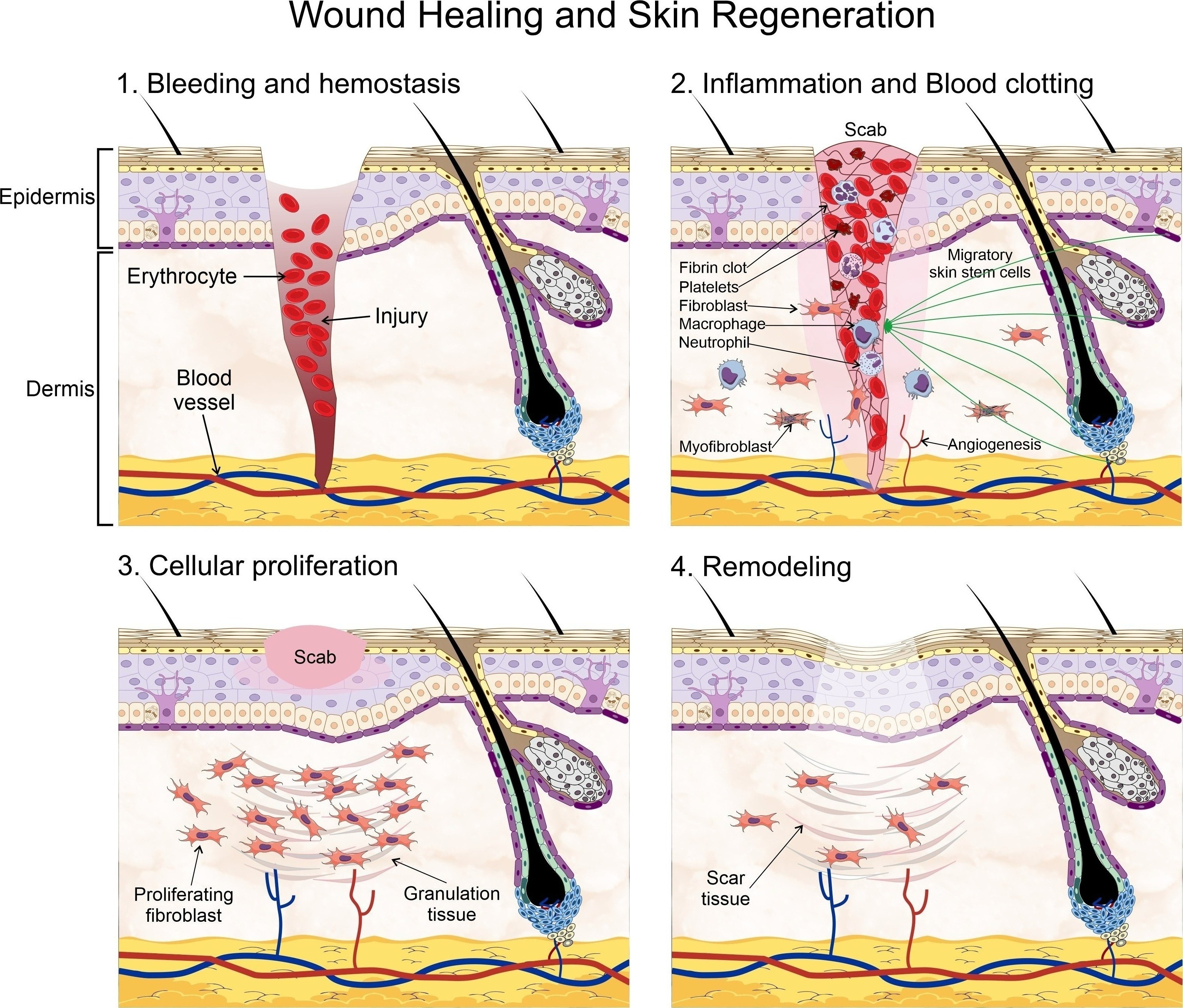
Figure 2. Wound healing process. Wound Healing and Skin Regeneration. Wound healing is a highly conserved mechanism that includes processes such as: 1. bleeding and hemostasis, in which blood flow slows and a clot forms to prevent blood loss during an injury, 2. Inflammation and blood clotting, in which the wound becomes sealed by fibrin which forms a temporary matrix occupied by immune cells whose task is to remove dead tissue and control infection, fibroblasts are then recruited into the site of the injury and promote angiogenesis and the recruitment of fibroblast-derived myofibroblasts, which contract the wound area. Skin stem cells are mobilized into the site of injury at this stage to begin the process of re-epithelialization starting from the edge of the wound, 3. Cellular proliferation, in which the recruited fibroblasts secrete collagen, and form granulation tissue, a scab is formed on the site of injury, and 4. Extracellular matrix (ECM) remodeling, in which new ECM components are secreted by both fibroblasts and epidermal keratinocytes, which also remodel the matrix through the expression of matrix metalloproteinases. Some elements of this figure were taken from the Mind the Graph platform, available at
www.mindthegraph.com (accessed on 27 September 2021).
2.3. Scarless Wound Healing
The formation of fibrotic scar tissue can be an unavoidable consequence of wound healing and, sometimes, it can result in severe cosmetic and psychological consequences. Normally, acute wound repair occurs only in response to severe injury; interestingly, this process is not directly driven by the SSCs present in the HF bulge. Instead, short-lived transient amplifying cells (TACs) are created for the healing process
[41]. Only slow-cycling stem cells and committed progenitor cells residing within the interfollicular epidermis (IFE) have a substantial contribution toward the repair and long-term regeneration of the damaged tissue
[42]. As previously mentioned, there are different and highly heterogenous stem cell pools in the skin, each with a specific role during wound healing. For instance, dermal papillae (DP) possess a mesenchymal cell population associated with HF growth and development, thereby constituting an important reservoir of multipotent stem cells
[43]. Other cells, designated as skin-derived precursors (SKPs), also contribute to skin maintenance, wound healing, and HF morphogenesis. Due to their self-renewing and multipotent capacity, these cells are considered as SSCs
[44].
While the role of SSCs in wound healing cannot be understated, relatively recent research has demonstrated the essential role of dermal fibroblasts in wound healing and scarring. These fibroblasts are usually classified into two main lineages according to their localization in either the upper or lower dermis, the former of which contributes to re-epithelization and hair follicle formation, whereas that the latter synthesizes the fibrillar extracellular matrix (ECM) in the reticular dermis and are heavily involved in dermal repair after injury, infiltrating the wound bed during healing. These fibroblasts express α-smooth muscle actin (α-SMA), thus presenting a myofibroblastic phenotype
[45]. These fibroblast lineages have a different response to Wnt/β-catenin signaling and, instead, Hedgehog (Hh) signaling promotes the proliferation of papillary fibroblasts whereas that TGF-β regulates ECM remodeling in the reticular dermis
[46]. It must be noted that two different pools of reticular fibroblasts, Sca1+/CD26+ and CD26+/En1+, constitute the majority of all dermal fibroblasts and are responsible for the connective tissue deposition leading to scar formation
[46][47].
Curiously, scar formation is absent during the early gestational period of mammals
[48]. The mechanisms involved are currently unknown; however, it may be possible that the existent differences between the developing skin and that of an adult, such as the decreased tensile strength of the early fetal skin, could play a role in this process. In this regard, the focal adhesion kinase (FAK) has been linked with fibrosis through the expression of extracellular-related kinase (ERK), which induces the secretion of monocyte chemoattractant protein-1 (MCP-1), a protein associated with diverse fibrotic disorders
[49][50]. Interestingly, the inhibition of the inflammatory FAK-ERK-MCP-1 pathway attenuates scar formation
[49]. The scar-free healing process observed in fetal tissues has been strongly associated with a reduced inflammatory response; in contrast, the healing of pathologically compromised injuries often involves an unregulated inflammatory process
[51]. Therefore, it may be surmised that the inclusion of anti-inflammatory agents could push the wound healing process toward a regenerative pathway instead
[52]. In this regard, the fibroblast growth factor 9 (Fgf9) can modulate the inflammatory response
[53] and promote the regeneration of the HF, which are normally lost when scarring occurs
[54]. The administration of interleukin 10 (IL-10) also contributes toward the regeneration of normal skin structures and attenuates the expression of pro-inflammatory molecules
[55]. On the other hand, the inhibition of the chemokine receptor type 4 (CXCR-4) prevents the recruitment of pro-inflammatory cells towards the wounded area and the activation of fibroblasts, thus resulting in scarless healing
[56]. Additional studies have identified diverse cell populations that contribute toward the formation of scars. One of such populations express disintegrin and metalloproteinase domain-containing protein 12 (ADAM12), both of which are involved in fibrosis and scarring
[57]. En1-derived fibroblasts have also been involved in cutaneous scarring as they are major contributors of connective tissue during scar formation
[47]. Myofibroblasts play a key role in normal wound healing by promoting the contraction of the wound and ECM production
[48]. Myofibroblasts are naturally heterogeneous and their population is known to include fibroblasts, mesenchymal stem cells (MSCs), smooth muscle cells, endothelial cells, and fibrocytes
[58]. Lastly, adipocytes have also been found during wound healing, which, apparently, are required for the recruitment of fibroblasts into the wounded area
[59].
Unequivocally, the regeneration capacity of the skin is driven by stem cells; therefore, stem cell-based therapies for wound healing are mostly aimed at recalcitrant wounds, although their capacity for scarless healing is still debated. The ability to regulate the wound environment is key for stem cell-based therapies, as they can downregulate the inflammatory response
[60][61][62]. Regrettably, the extensive application of stem cells in wound healing therapy is restricted by practical limitations
[63]. Regardless, other strategies seem promising; one of such involves the use of umbilical cord (UC)-MSC conditioned media, which can induce dermal fibroblasts to behave like fetal fibroblasts, e.g., lower myofibroblast differentiation, diminished TGF-β1/TGF-β3 ratio, and increased ECM remodeling. Unsurprisingly, skin injuries treated with UC-MSC conditioned media heal faster and accumulate less collagen
[64]. Other studies with MSC derived from human amniotic fluid have shown great potential to inhibit the pro-fibrotic activity of TGF-β1 as well as the ability to reverse the phenotype of myofibroblasts back into a fibroblast-like state.
Wound healing has been linked to great extent with Wnt signaling, of which the canonical pathway (Wnt/β-catenin) plays a prominent role in skin regeneration and repair. Since this pathway has already been extensively described in other reports, we will only provide a brief recapitulation of its involvement in wound healing. The levels of β-catenin are remarkably high during normal wound healing with scar formation
[65] and other studies have shown a clear association between TGF-β and the Wnt/β-catenin pathway during fibrosis, e.g., hypertrophic scars and keloids
[66]. Moreover, human keloids display an upregulated Wnt-3a expression, inducing the transition of endothelial fibroblasts to mesenchymal cells, and thus to the accumulation of collagen
[67]. On the other hand, TGF-β signaling is crucial for a pro-fibrotic fibroblast phenotype, which also involves the strong participation of the Wnt pathway. In the absence of β-catenin, TGF-β1 cannot promote hyperplastic wounds in mice
[68]. TGF-β also downregulates the Wnt-antagonist Dkk-1, thereby upregulating the Wnt/β-catenin signaling pathway
[69]. The application of Wnt pathway inhibitors leads to the formation of rete pegs and dermal papillae after wound closure, which would be otherwise lacking under normal healing conditions with scar formation. Regarding the latter, its formation can also be prevented by inhibiting the Wnt pathway
[70]. Its apparent efficiency notwithstanding, a careful balance must be struck in this regard as the inhibition of β-catenin can also lead to decreased contraction, migration, and collagen production, thus having an overall negative effect on wound healing
[71]. On the other hand, the activation of epidermal Wnt/β-catenin signaling can promote the expansion of upper dermal fibroblasts enabling HF growth, which is typically absent under normal wound healing conditions
[45][48]. Therefore, upper dermal fibroblasts are currently considered as a plausible alternative in the promotion of scarless wound healing
[72].
2.4. Chronic Wounds
As we already know, wound healing is a complex and multi-step procedure that requires an inflammatory reaction, wound clotting, re-epithelialization, remodeling regulation, and stem cell control. In recent years, the role of SSCs in wound healing has been recognized as an integral part in the healing of diabetic wounds, as they are essential during the steps of wound closure and tissue remodeling. Therefore, the direct loss of SSCs, their impaired migration capacity, and/or faulty differentiation capacity may have a profoundly negative effect on wound healing. One of such impairing effects has been directly related with telomere stability, as it can hinder both the proliferation and migration capacity of SSCs [73]. The telomeres have been involved in a wide range of cellular processes, including ageing and cancer. Moreover, telomere maintenance is crucial for the longevity of stem cells. The upkeep of the telomeres’ length is responsibility of an enzyme known as telomerase, which is normally downregulated in somatic cells but highly expressed in both cancer and stem cells. An integral part of this enzyme is known as telomerase reverse transcriptase (TERT) [74]. TERT is involved in some types of trauma, particularly through the activation of NF-κB and autophagy [75]. In SSCs, however, TERT expression is required for normal cell proliferation and migration; further, its expression is closely associated with the activation of the Wnt/β-catenin signaling pathway [76]. The inactivation of this signaling pathway, which has been associated with the inflammatory response in diabetic ulcers, wound proliferation and remodeling [77], has also been linked with the expression of telomerase; although the precise mechanism still remains obscure.
2.5. Wound Healing Therapy
Cultured epidermal cell sheets (CES) have been used in the treatment of non-healing wounds with relative success and, while they do indeed help to save lives, the normal function and appearance of the skin after the transplant is still in need of improvement. Therefore, several synthetic skin substitutes have been developed to enhance the function, strength, and integration capacity of the transplanted CES. In this regard, it was discovered that enriching these CES with SSCs results in a better outcome regarding function
[78], scar formation
[79], and long-term regeneration of the skin
[80], and it has been proven that CES transplants in severely burned areas increase patient survival
[81][82]. To provide further insight into this fascinating issue, we highly recommend the work of Jang at al.
[83] and Jackson et al.
[84] as additional reading material. Regardless, there are still serious issues, such as engraftment deficiency, appearance, and function, which remain to be solved
[85]. Other therapeutic approaches involve the use of synthetic skin substitutes consisting of a matrix that can be seeded with cells; however, these allografts are not without limitations, including faulty engraftment
[86]. Other limitations include their simplistic structure, which is unable to recreate the native conditions needed for cell signaling
[87]. Newer skin substitutes are formed by composite layers compatible with the ECM, several cell types, and ECM-bound growth factors
[88]. Enriching these skin substitutes and CES with SSCs before transplantation may have an improved outcome, including the successful engraftment of the cultured SSCs
[80]. However, the variable number of SSCs per patient, along with non-standardized protocols for their culture and phenotype preservation, have become important factors in the failure of these transplants. Therefore, the use of integrin β1-compatible substrates that can also mimic the SC niche is being implemented to improve the clinical outcome of these therapies.