Personalized point-of-care testing (POCT) devices, such as wearable sensors, enable quick access to health monitoring without the use of complex instruments. Wearable sensors are gaining popularity owing to their ability to offer regular and continuous monitoring of physiological data by dynamic, non-invasive assessments of biomarkers in biofluids such as tear, sweat, interstitial fluid and saliva.
1. Introduction
Regular and real-time monitoring is required for improved care of individuals suffering from chronic illnesses such as cardiovascular disease, diabetes and neurological disorders. Chronic diseases, according to the World Health Organization (WHO), account for three-quarters (75%) of all deaths worldwide and impose significant economic burdens
[1][2][3][4]. Personalized point-of-care testing (POCT), a quickly progressing area in clinical testing, is evolving as a current diagnostic procedure for examination, testing and other medical applications
[5][6][7][8]. The era of POCT began in 1962 with the development of a new, rapid method for measuring blood glucose levels, and it was further progressed in 1977 with the advent of a rapid pregnancy test
[9][10]. POCT in clinics or hospitals gained attention in the early 1990s with compact, portable devices capable of assessing several electrolytes of patients in emergency rooms. With the focus of healthcare changing toward disease prevention and early detection, as well as chronic condition monitoring, there is an increasing demand for painless, patient-centred sensor technology
[11][12][13][14][15][16]. Portable devices have proven useful in diagnosis and monitoring of various health conditions, including commercialized blood glucose monitoring. Wearable sensors (WS) with continuous monitoring capacity, on the other hand, have progressed from monitoring of generic physiological biomarkers (e.g., pressure and temperature) to much more specific purposes such as diabetes management and other diseases. The advancement of flexible, elastic and electronic technology has also allowed a wide range of wearable devices for clinical diagnostic and monitoring in the individual medical field. Although not all WS for POCT have to be flexible or stretchable, those having increased deformability and conformality offer good options for developing a revolutionary next-generation WS for POCT
[17]. Moreover, the incorporation of synthetic biology into wearables may broaden the possibilities for non-invasive surveillance of physiological statuses and exposure to infections or poisons
[18]. WHO’s REASSURED framework during technology development includes strategies for the manufacture of a perfect POCT device. REASSURED includes real time connectivity, ease of specimen, affordability, sensitivity, specificity, user friendly, rapid and robust, equipment free and deliverable to end users
[19][20][21]. Modern WS can take high-quality measures on par with controlled medical devices. As a result, the line among customer and clinical wearable gadgets is becoming less.
First-generation WS, such as shoes, watches or headsets, were primarily aimed on biophysical measuring, such as tracking an individual’s physical activity, heart rate or temperature of body
[22][23]. By the widespread adoption of first-generation wearables, attention has gradually shifted to the development of a non-invasive or minimally invasive biochemical and multifunctional monitoring device, which is the subsequent stage towards personalised health care
[24]. The features of wearable systems have been altered in past years, with scientists shifting their focus away from monitoring physical exercise activity and toward tackling important challenges in healthcare applications such as diabetes treatment and surveillance systems of the elderly. To achieve these objectives, researchers have made significant investments in the fabrication of wearable systems that are sensing devices which integrate a biological recognition aspect into the operation of a sensor
[25]. The constantly growing rate of a new disclosed proof-of-concept research demonstrates the possible utility of WS. Several commercially available hand-worn sensors for tracking physical activity, such as Apple Watch and Fitbit, have now become progressively more popular over the general population. A number of constant glucose tracking devices have also entered the shops (for example, Medtronic’s Guardian Real-Time and Abbott’s FreeStyle Libre). One especially appealing category of mobile health sensors is bioaffinity sensors, which use a ’bio recognition’ component for high affinity of the target sample
[26][27]. The inclusion of bioaffinity components with increasing selectivity and sensitivity for the identification of disease targets will expand the WS landscape and the effect of digital health. Although several of these technologies are in clinical trials, successful commercialization has proved elusive. Significant efforts are being made to commercialise non-invasive sensors. These products, however, still need large-scale evaluations, device regulatory approvals and last marketing strategies.
Further, Internet of Things (IoT) is a potential approach for providing regular, accurate, and holistic monitoring, reducing human labour and support in medical decision making. IoT is a novel idea which permits healthcare tracking using wearable devices. It is a system of physical items that have embedded techniques for the detection and interaction with the environment, and for providing autonomous communication. WS are a prominent IoT application field that has received a lot of consideration in the previous decade, owing to the fact of inexpensive fitness applications in the market sector.
2. Wearable Point-of-Care Testing for Self-Health Care
Rapid advancements in sensor technology have resulted in the creation of high-performance WS for use in self-health monitoring. This expansion of WS can be attributed to a diversity of factors, such as the accessibility and ergonomics provided by advances in miniaturised electronics, the expansion of smartphones and smart devices, an increasing customer desire for awareness campaigns and the unmet need for doctors to obtain medical quality data from their patients on a continuous basis
[28]. Despite this early accomplishment, there is still a strong desire to acquire even more metabolic data from the human body. WS are an outstanding alternative for medical applications because of their advantageous characteristics. They are designed, manufactured, and used in self-health care systems to aid in diagnosis of various diseases. Specific parameters, such as heart rate, pressure or temperature, can be tracked and evaluated depending on the type of sensor. Wearables provide a novel arena for monitoring of health and testing in which persons require no expertise and can monitor and evaluate their health condition intermittently or continuously on routine
[29][30]. Wearable sensors can be physical, where the sensor measures the electrophysiological activity
[31] (electrocardiograms, electromyograms or electroencephalograms) and physiological conditions (heart rate, temperature
[32] and movement
[33][34]) or biochemical, which is used to monitor body fluids by non-invasive or slightly invasive sample collection from body parts such as skin, saliva, mouth, etc., that will ultimately help in determining the state of health of the person. For continuous monitoring without any data transfer, a reader element is incorporated on the on-body gadget. The sensing component is usually colorimetric in wearable devices based on a biochemical sensor that uses optical transduction of a signal
[35]. The change in colour is taken with the help of a photographic camera and transferred to a data reader, where the analysis is performed by software, that calibrates and displays the results.
Scheme 1 represents the overall view of the wearable sensors (sampling, types) and their applications.
Scheme 1. An overview of design, types and applications of wearable sensors.
3. Design of Wearable Sensors
3.1. Sampling Methods
The method of collecting a sample plays a vital part in the analysis of WS for POCT for self-use. The sampling method must be simple and easy, optimal storage conditions and its transport, no contamination and reduced intervals between sampling. The samples for these sensors include sweat, saliva, interstitial fluid (ISF), blood or wound fluids.
3.2. Sensor Materials
The basic requirement for the fabrication of wearable sensors includes stretchable, mechanically flexible, biodegradable and should adapt with the movement of human body. Poly (vinyl alcohol), poly(dimethylsiloxane) (PDMS), Ecoflex, polyurethane (PU), poly (ethylene terephthalate) (PET), polyester etc are some of the materials that are commonly used as sensor materials
[17][18]. Similarly, several nanomaterials (organic, inorganic or hybrid) are also widely used as sensing materials
[8]. Further, based on the type of sensor, the property of the material used also varies. For example, in case of sensors implanted in the body, biocompatible and biodegradable materials are used.
3.3. Sensing Approaches
Wearable devices for sensing applications in sweat, blood, ISF etc have been designed by means of signal transduction methods such as electrochemical and optical signal. Colorimetry is the well-studied optical transduction approach in WS because of its minimal price, simplicity, and automated operation. Usually, in this type of sensors conventional dyes are combined with enzyme, ion indicators, or mesoporous resin beads on the surface of polymer or filter paper to create a layer for sensing
[36]. The generation of novel nanomaterials with outstanding electrical and optical properties, capable to detecto all metabolic analytes (e.g., glucose, lactose) with high sensitivity and better stability are required for the fabrication of colorimetric wearables
[37]. Further, wearable optical sensors which utilize fluorescence or SPR for the optical transduction method demonstrated to promote the sensitivity and specificity of the analytes
[37]. Another possibility for wearable biochemical sensing is electrochemical method. Electrochemical enzymatic wearables are the most frequent used as they have unique benefits in compactness, excellent selectivity, and label-free direct monitoring. Yet, some limitations of enzymatic WS include less stability, limited sensitivity, weakness to variations in environmental parameters such as temperature, humidity and pH, and difficult manufacturing methods
[38][39].
3.4. Signal Processing Unit and Power Supply
Sensor signals must be correctly obtained and transferred to a device for processing, analysis, and display externally. For these purposes, electronic circuit boards have been established. Still, at the wearable-system level, processing, evaluation, data processing, and display of signals must be smoothly linked. Signal processing circuitry is often on the basis of integrated-circuit chips that is available in the market, but all of those components of chip are stiff, preventing the seamless incorporation at the wearable-system level. Further, the creation of self-driven sensors is critical for the progress of POCT systems because it allows for power saving in a fully integrated wearables or in the building of an independent POCT device without external power supply. Biofuel cells (BFC) have the potential to be self-driven since it can be feasibly engineered to function as self-power-driven electrochemical sensors by presenting a signal proportionate to the amount of target biomolecule in the sample
[40][41]. The initial WS based on self-driven concept was developed by incorporating a BFC on a glucose biosensor device inside a contact lens for detecting the level of glucose in tears
[42]. Further, the integration of biofuel cell with near field communication (NFC) enabled platform is one of the emerging means to operate a fully integrated WS
[43][44]. A wireless sweat glucose sensor that operates battery free was developed on a microfluidic platform with NFC electronic module. The system was attached on the skin for the regular tracking of the concentration of glucose and lactate
[45]. Similarly, a wireless, battery free, microfluidic based sweat sensor was also developed (
Figure 1).
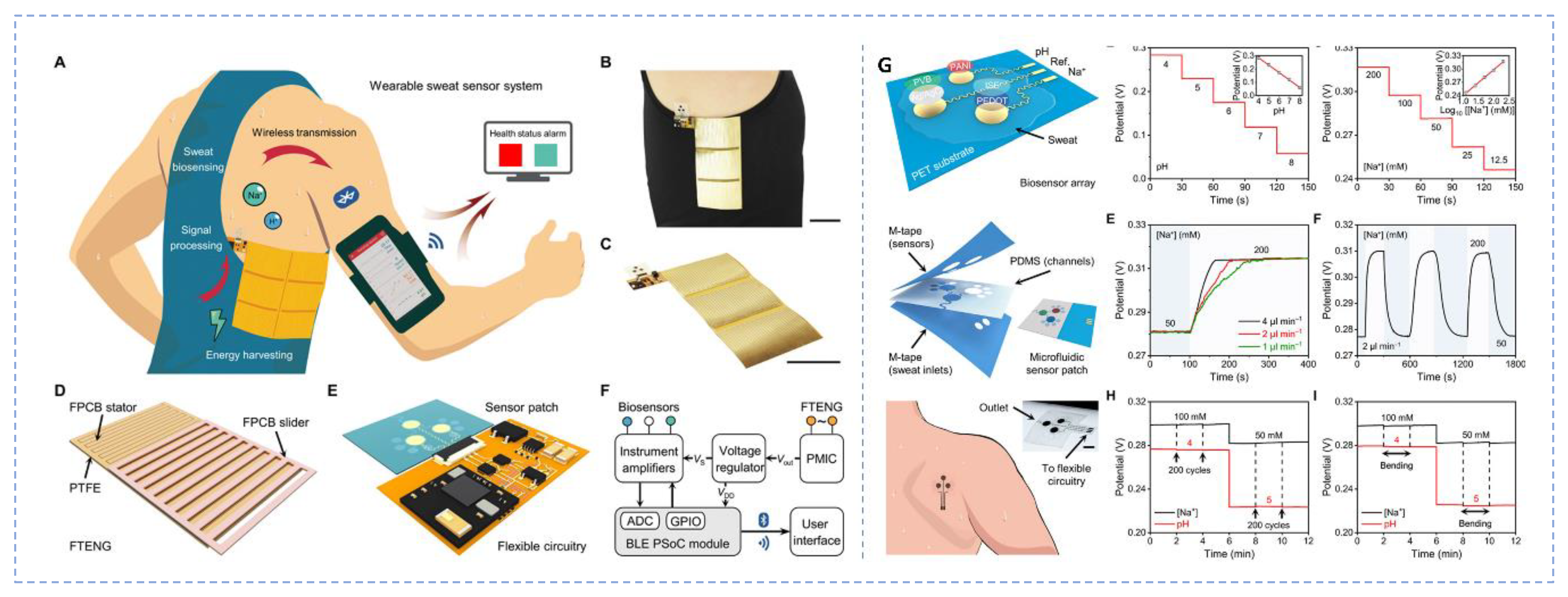
Figure 1. A microfluidic based wireless battery free sweat sensor (
A). Freestanding-mode TENG (FTENG) wearable sweat sensor system (FWS
3) for real time sweat biosensing; (
B,
C). Images of the sensor worn on side torso of human; (
D). Flow chart of flexible printed circuit board based FTENG with a grating slider and an interdigital stator; (
E). Microfluidic-based sweat analysis patch interfacing with the flexible circuitry; (
F). Working process of the sensor; (
G). Flexible biosensor array comprising a pH sensor and a Na
+ sensor patterned on a flexible PET substrate and their calibration plots. (Reprinted from
[34] which is an open access article distributed under the Creative Commons Attribution License).
4. Types of Wearable Sensors
Based on a variety of aspects, including the design and utility, materials utilised, signal transduction method, nature of analyte or signal etc., WS were grouped into many types
[46]. WSs can be categorised as wearable bands (watches and gloves), wearable textiles (t-shirts, socks, and shoes), wearable gear (spectacles and helmets), and sensory systems for tracking, based on their design and utility. The WS performance and wear resistance can be improved, and its usefulness can be increased, if the suitable material is available. WSs can be divided into three types based on the materials: biodegradable flexible sensors (rice-papers, nanofibers, fibroin), wearable biocompatible sensors (cellulose, chitin, alginate) and self-healing flexible sensors (hydrogel)
[47]. WSs are widely divided into biophysical, biochemical, and multiplexed sensors based on the signal. Mechanical (strain, pressure, vibration, and tactile), thermal (fever), and electrophysiological (ECG, EEG, EMG and EOG), biophysical biosensors are additional subcategories of biophysical biosensors. Health-related signals such as glucose, cortisol, pH, cytokines, gas (alcohol gas sensors), hormone, nutrients, and others are picked up by biochemical sensors
[48]. Biological inputs such as bacteria, cells, hormones, tissues, enzymes, chemical receptors, and other analytes that comprise antibodies, nucleic acids, and immunological agents can be detected by using either biochemical or multiplexed WS. Wearable sensors (worn on the head, neck, chest, legs, feet, arms, and fingers) are made possible by the range of transducing mechanisms that are currently accessible. A crucial element in transforming various biosensing technologies into wearable gadgets is the design of transducing mechanism.
4.1. Wearable Colorimetric Sensors
A wearable colorimetric sensing platform that included sensor patches with bromocresol green pH indicator dye in a closed headspace over the skin was studied. When basic volatile nitrogen molecules such as ammonia and amines are released from skin, the sensor spots’ colour changes
[49]. The glucose oxidase (GOD)-peroxidase-o-dianisidine reagents were developed as a microfluidic chip-based wearable colorimetric sensor for detecting the glucose amount in sweat. It was discovered that the colour shift brought on by the enzymatic oxidation of o-dianisidine. The obtained linear range for sweat glucose was 0.1–0.5 mM with a detection limit of 0.03 mM
[50].
4.2. Wearable Mechanical Sensors
High-performance wearables need strong, stretchable fibres. Fibrous materials with increased mechanical strength and tensile property are difficult to make. Ultra-robust (17.6 MPa) and extensible (700%) conducting microfibers are produced and used to make fibrous mechanical systems. The mechanical sensor is sensitive to strains with excellent resolution and a wide detection range (0.0075% to 400%). Low-frequency vibrations between 0 and 40 Hz cover most bodily tremors
[51]. For the purpose of detecting mechanical deformation, wearable auxetic materials based on ionogel and metal-organic frameworks were created using 3D printing. In order to track different human body movements, the resulting auxetic sensor displayed excellent sensitivity through a change in resistance following mechanical deformation with skins
[52]. A quick-resilient, hysteresis-free, vinyl hybrid silica nanoparticle (VSNPs), polyacrylamide (PAAm), and alginate double-network hydrogel-based strain sensor was created by dynamically cross-linking the PAAm network to preserve the hydrogel’s integrity.
4.3. Wearable Electrochemical Sensors
Wearable electrochemical sensors provide a lot of potential for continuous, non-invasive, regular monitoring of analytes and full health evaluation. The monitoring of phenylalanine (PHE) using a wearable wristband electrochemical sensor has been described. To eliminate interferences in biofluids, the suggested electrochemical sensor is built over the screen-printed electrode (SPE) modified with a membrane made of Nafion. In order to derivatize PHE
in-situ into an electroactive product and enable its electrochemical oxidation at the surface of SPE under alkaline circumstances, the membrane additionally contains sodium 1,2-naphthoquinone-4-sulphonate
[53]. Flexible reference electrode, pH response electrode, and K
+ selective electrode that made up the sensor were made by printing an aqueous suspension of β-CD (cyclodextrin) functionalized graphene (β-CD/RGO) on a conductive substrate using an electronic printer
[54]. For constant tracking of the level of glucose in sweat with great sensitivity, a wearable electrochemical sweat sensor based on a Ni-Co MOF nanosheet covered Au/polydimethylsiloxane (PDMS) film has been developed
[55].
4.4. Wearable Optical Sensors
Li
2ZnSiO
4:Mn
2+ is incorporated into stretchable elastomer-based optical fibres to create a wearable optical temperature sensor. This material can offer thermal-sensitive emissions at dual wavelengths for steady and constant ratiometric temperature monitoring that has better accuracy and repeatability
[56]. A sensitive non-enzymatic fluorescence sensor for glucose detection can be made using printed circuit board substrates made of vertically aligned ZnO nanotubes (NTs). The sensor’s performance is determined by the quenching of photoluminescence (PL) in ZnO NTs treated with varied quantities of glucose. The sensor’s sensitivity is 3.5% mM
−1 (percentage change of the PL peak intensity per mM), and its lower limit of detection (LOD) is 70 μM
[57].
5. Application of Wearable Sensors for Self-Health Care
5.1. Temporary Tattoos Integrated with Sensor
Temporary tattoos possess certain mechanical properties alike to body skin and may be effortlessly utilised for the real time connection in spite of repetitive mechanical twist, making them ideal for WS and POCT devices
[58]. Wang’s group, in 2013 created the first tattoo-based biosensor, which was amperometric and was made by screen printing directly on the working, reference and counter electrode into a tattoo and attaching it to the skin
[59]. The device was successfully tested in humans for constant monitoring of lactate in sweat during cycling. Similarly, a tattoo-based sensing system was fabricated for glucose monitoring at rest state. This was the first wearable tattoo sensor that combined reverse iontophoretic method for the collection of glucose in the ISF and an enzyme based amperometric biosensor
[60]. Conventional screen printing and solid contact ion selective electrode method was used for the fabrication of a tattoo that can monitor the epidermal pH level from human perspiration real time during an active physical activity
[61].
5.2. Accessories Integrated with Sensor
Recently, wearable accessories in the kind of watches, spectacles, mouth guards, and contact lenses, are intended to analyse perspiration, ISF, tears, and saliva. These accessories are incorporated with the ability for signal detection and data processing elements (such as potentiostats, microcontrollers, and bluetooth wireless communication modules) to regulate operation of system, translate analogue signals to digital signals, analyse digitalised information, and transfer data to off-body system for regular, on-body tracking. Field effect transistor based wearable smart watch-based sensor was developed for the detection cortisol in sweat and saliva samples
[62].
6. Conclusions
Wearable sensors with novel structural designs paired with functional micro/nanomaterials enable the continuous tracking of a medical status at both physiological and biochemical levels. Wearable sensors are promising and have the potential to revolutionise therapies and diagnosis by altering the method of data collection, processing and analysis. Possible applications are numerous and are predicted to expand significantly as they get integrated into everyday wearables. WS integrated with nano-diagnostic systems have been effectively utilised for the identification of samples varying from different biomolecules such as proteins, hormones and nucleic acids) to infectious disease-causing microbes. However, more studies on the in-depth investigation of the compositions of diverse biofluids is required for identifying previously unknown biomarkers. Further, most analyte concentrations are not the same in biofluids as they are in blood, hence, establishing a good correlation between the composition of biofluids and blood chemistry is important for any future commercial applications.
This entry is adapted from the peer-reviewed paper 10.3390/diagnostics13050916