1. Introduction
Phenolic compounds are components that contribute to the bitterness, astringency, and pigmentation of most plants. In addition to providing color to flowers, the physiological role of these compounds in plants is to confer biological protection against damage caused by ultraviolet rays, feeding by insects and herbivores, and pathogenic microorganisms. The type of phenolic compounds is dependent on its chemical structure [1] and includes well-known “catechins”, “isoflavones”, and “anthocyanins”. Phenolic compounds and their analogs have a wide variety of molecular sizes and structures (Figure 1).
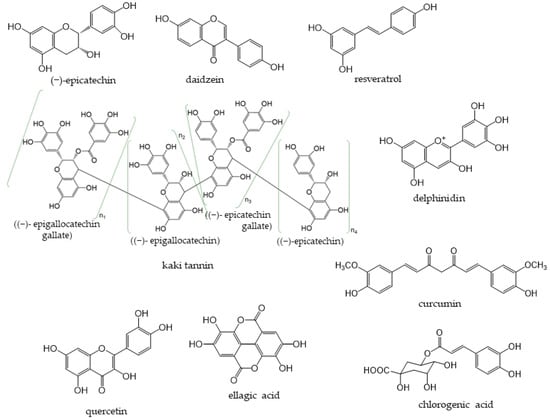
Figure 1. Representative phenolic compounds.
Previous studies on the antioxidant activity of phenolic compounds confirmed their role in the detoxification of excess reactive oxygen species (ROS) and the prevention of lifestyle-related diseases. The biological effects of phenolic compounds depend on the amount consumed and their digestion, absorption, and bioavailability. The majority of these compounds are not absorbed in the small intestine and reach the colon, in which glycosides are hydrolyzed and degraded by intestinal bacteria, generating various catabolites [2]. These catabolites have been found to contribute to human health.
Among health issues, lifestyle diseases and neurodegenerative diseases are of great concern. As a dietary method that contributes to health, there is a ketogenic diet that mainly consists of lipids which is useful for Alzheimer’s disease relief [3] or prevention of obesity and diabetes [4]. In addition to these kinds of diet, dietary phenolic compounds and their catabolites also have health benefits in cardiovascular diseases [5], rheumatoid arthritis [6,7], depression [8,9], and eye diseases [10].
2. Flavan-3-ols
Flavan-3-ols (flavanols) are a group of flavonoids that have a 2-phenyl-3,4-dihydro-2H-chromen-3-ol skeleton. Dietary flavan-3-ols are abundant in cocoa, tea, apples, grapes (including red wine), berries, plums, apricots, and nuts. Flavan-3-ols are complex flavonoids in which monomers, such as catechins and epicatechins, make up units to form oligomers and polymers. They are components of proanthocyanidins, and many analogs exist in nature. Catechins, major dietary monomers, are abundant in tea leaves, and many studies have investigated their antioxidant properties [11,12]. Unlike other classes of flavonoids, flavan-3-ols are not present in a glycosylated form in foods [13] and monomeric flavan-3-ols are exceptionally absorbed in the small intestine. The galloylation and polymerization of flavan-3-ols were shown to significantly delay intestinal absorption [14]. Therefore, when oligomers and polymers reach the colon, they need to be metabolized by the colonic microbiota to provide health benefits.
The mechanisms underlying the antioxidant effects of monomers have been reported [15]. The antioxidant capacity of flavan-3-ol monomers is exerted through phenolic hydroxyl groups that trap ROS and the chelation of iron ions to prevent lipid peroxidation [16,17]. By indirectly employing antioxidant pathways, flavan-3-ols regulate the synthesis of antioxidant-related enzymes and the signaling pathways of oxidative stress [18]. However, the mechanisms of action of oligomers and polymers remain unclear.
2.1. Dietary source and metabolism of flavan-3-ols
2.1.1. Tea
Tea is a major source of catechins. Various types of tea are available from the Camellia sinensis (L.) plant, depending on the harvesting and processing of its leaves. Green tea is unfermented tea; black tea is completely fermented tea; white tea and oolong tea are tea types with different degrees of fermentation [19]. There are five main types of catechins present in tea: (+)-catechin, (−)-epicatechin (EC), (−)-epigallocatechin (EGC), (−)-epicatechin gallate (ECG), and (−)-epigallocatechin gallate (EGCG) (Figure 2) [20,21]. EGCG is the most abundant catechin in unfermented teas (green tea and white tea) [22]. During the fermentation of black tea, catechins are oxidized by polyphenol oxidase to complex structures, such as theaflavin dimers and thearubigin polymers [23]. Tea phenolic compounds and their metabolites possess antibacterial properties against pathogenic bacteria, such as Clostridium perfringens, C. difficile, Escherichia coli, Salmonella, and Pseudomonas species, and enhance the activities of probiotics, including Bifidobacterium and Lactobacillus species, thereby improving the overall balance of intestinal microbes [24,25].
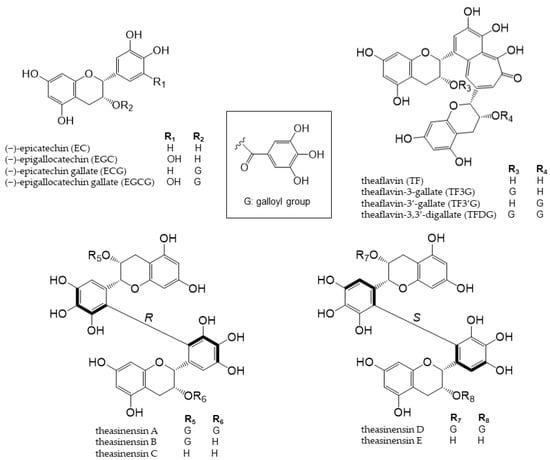
Figure 2. Chemical structures of catechins, theaflavins, and theasinensin A–E.
Theaflavins and theasinensins are catechin dimers that are not absorbed in the small intestine to the same extent as catechin; they reach the large intestine and are metabolized by intestinal bacteria enzymes [26–28]. Four theaflavins exist in black tea: theaflavin (TF), theaflavin-3-gallate (TF3G), theaflavin-3’-gallate (TF3’G), and theaflavin-3,3’-digallate (TFDG) (Figure 2), with TFDG being the most abundant [29]. TFDG alters the composition of the intestinal flora, similar to EGCG; however, the metabolic profile was significantly different [28]. The accumulation of further findings from in vivo studies is expected. Theasinensins are also catechin dimers with two galloyl groups; five theasinensins in fermented tea have been identified and named theasinensins A, B, C, D, and E (Figure 2) [30]. Theasinensin A is the most abundant among the five compounds [27]. The galloyl group is easily removed by intestinal bacteria and decomposed into theasinensin C. However, the progression of the subsequent reaction is slower than that of EGCG, and the whole picture remains unclear. In vivo studies are needed on these compounds, and the findings obtained will contribute to human health [27].
2.1.2. Cocoa
Cocoa is generally produced by fermenting and roasting the seeds of Theobroma cacao and then pulverizing the cocoa cake obtained by removing the fat content. Although flavan-3-ols are relatively abundant in cocoa, its components vary depending on the type of cacao, place of origin, time of harvest, and processing of cocoa [31–33]. Cocoa flavan-3-ols, along with (+)-catechin and procyanidin B1 and B2 (Figure 3), as well as trace amounts of other flavanols [34], mostly exist as EC.
Phenolic compounds in cocoa are metabolized in both the small and large intestine to produce metabolites that affect human health. [31,35,36].
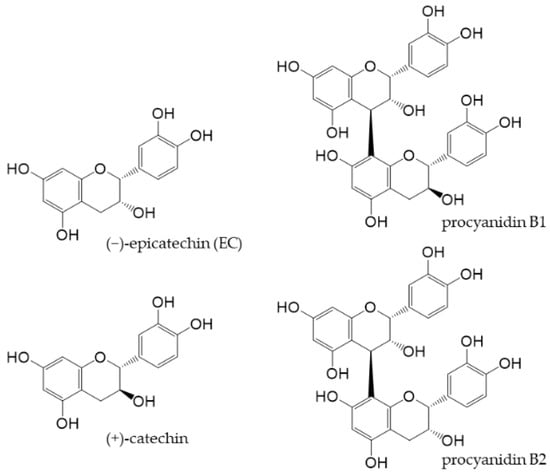
Figure 3. Chemical structures of main flavan-3-ols in cocoa powder.
2.2. Health benefits of flavan-3-ols
2.2.1. Tea
A well-established causal relationship has been reported between the intake of EC and the regulation of cardiovascular function [37,38]. EC is rapidly absorbed, and its metabolites are excreted in the urine 72 h after consumption [39]. Although EC does not affect the composition of the microbial flora[40], EC phase II and gut microbiota metabolites may induce complex nutrigenomic/epigenomic changes that regulate the function of brain endothelial cells[39,41]. In other words, the metabolites of EC may reduce the risk of neurodegenerative diseases by maintaining the integrity of cerebrovascular endothelial cells, suggesting that the intake of EC contributes to improvements in cognitive ability[41].
The ingestion of tea reportedly attenuates alcoholic liver disease [42]. The administration of tea extract has been shown to activate antioxidant enzymes in the liver, change the intestinal flora, and promote liver function [43,44]. Although some types of teas promote liver function, others exert the opposite effects; therefore, further research on this subject is required [42,43].
EGCG is the major catechin found in unfermented tea [19] and exhibits the highest antioxidant activity among the four catechin monomers in vitro [21]. EGCG may attenuate non-alcoholic fatty liver disease (NAFLD) by regulating the interaction between the gut microbiota and bile acids [45].
NAFLD is closely associated with the gastrointestinal microflora and its dysbiosis [46,47]; therefore, further research on the treatment and prevention of NAFLD is needed. EGCG reportedly prevents the occurrence of NAFLD by regulating the intestinal flora. Akkermansia muciniphila, belonging to the phylum Verrucomicrobia, has been implicated in obesity, glucose metabolism, and intestinal immunity [48]. The abundance of the genus Akkermansia has been shown to increase with the intake of phenolic compounds and exerts anti-obesity effects[49]. Furthermore, EGCG intake increased the abundance of the genus Akkermansia in mice compared to a high-fat diet [45].
Inflammatory bowel disease (IBD) is an inflammatory disease that collectively refers to ulcerative colitis (UC) and Crohn’s disease, which are generally considered to have unknown (non-specific) etiologies. Catechins exhibit anti-inflammatory, antioxidant, and antibacterial activities, which may improve the abnormal condition of intestinal bacteria caused by IBD [50–53]. However, depending on the doses of catechin examined, conflicting findings have been reported; therefore, further research on this subject is needed [18].
Catechins in tea are metabolized into phenyl-γ-valerolactones by the action of intestinal bacteria. Phenyl-γ-valerolactones regulate cellular proteolysis and exert neuroprotective effects [54]. Animal studies also revealed the beneficial effects of EGCG on neurodegeneration in animal models of Alzheimer’s disease [55] and Parkinson’s disease [56,57]. Furthermore, EGCG was shown to affect hypoxia-induced neuroinflammation in cell lines [58]. Based on these findings, the intake of catechin may be effective against neurodegenerative diseases.
2.2.2. Cocoa
Cocoa powder has been shown to affect the gut microbiota by changing their metabolites and promoting the growth of Lactobacillus and Bifidobacterium groups in pigs [59] Flavanols in cocoa may function as prebiotics to maintain intestinal immunomodulation by regulating the gut microbiota [60–62]. The ingestion of cocoa powder was previously suggested to change the intestinal flora of the diabetic Zucker rat model, by strengthening the intestinal barrier and ameliorating colonic inflammation, thereby attenuating diabetes [63]. Cocoa powder was also shown to down-regulate inflammation markers and suppress inflammation-related colon carcinogenesis; therefore, its consumption may be promising for the prevention of intestinal inflammation and related cancers [64]. Cocoa flavanols also exert endothelium-dependent vasodilatory effects [65], suggesting their potential to ameliorate cardiovascular diseases [66].
3. Condensed Tannins
Tannin is a general term for astringent plant components that exist widely throughout the plant kingdom and have been traditionally used to tan leather. There are two types of tannins, one of which is hydrolyzed tannins which are polymers of ellagic acids or gallic acids, and the other is condensed tannins which are polymers of catechins. They are hydrolyzed or decomposed under specific conditions and produce low molecular weight phenolic compounds. The astringent skin of chestnuts and walnuts contain hydrolyzed tannins and astringent persimmons contain condensed tannins. Red wine also contains condensed tannins, but the degree of polymerization of catechins are altered depending on the degree of fermentation and the manufacturing method.
Kaki tannins have also been reported to reshape the gut microbiota in rats fed a high-cholesterol diet [67].
Mycobacterium avium complex (MAC) is the most common nontuberculous mycobacterium that causes chronic pulmonary infections in immunodeficient individuals. Kaki tannins, used as a dietary supplement, reduce the symptoms of pulmonary MAC infection [68], suggesting an impact on mucosal immune inflammation, including that of the gut, through their anti-inflammatory effects and changes to the gut microbial composition. Moreover, kaki tannins may need to be digested and/or fermented into smaller molecules in vivo prior to their absorption into the body in order to exert their beneficial effects. The artificial digestion of the non-extracted residues of dried persimmons containing kaki tannins suggested that intestinal bacteria degraded the tannins into lower molecular weight fragments [69].
UC is a chronic IBD induced by the dysregulation of the immune response in the intestinal mucosa. The pathogenesis of UC was less severe in a mouse model fed kaki tannins than in a control diet group [70]. Furthermore, the gene expression of an inflammatory cytokine (IL-1β) and chemokine (CXCL1) was significantly decreased in the tannin diet group. An analysis of the composition of the fecal microbiota of mice employing 16S ribosomal RNA gene sequencing revealed that a treatment with DSS significantly increased the abundance of the phylum Enterobacteriaceae in the control diet group, whereas it was significantly suppressed in the kaki tannin diet group.
4. Flavonols
Flavonols, a subclass of flavonoids with a 3-hydroxyflavone skeleton, are widely present in plants [13]. Typical flavonols include myricetin (in grapes and berries), kaempferol (in tea, broccoli, and ginger), rutin (in asparagus and buckwheat), and quercetin (Figure 4). Quercetin is a representative flavonol that has been extensively examined and is present in vegetables and fruits, such as onions, broccoli, and apples. Flavonols generally exist in a glycosidic form and are deglycosylated and absorbed in the small intestine. After absorption, they are rapidly metabolized by phase II enzymes in the liver and circulate as methyl, glucuronide, and sulfate metabolites [71,72]
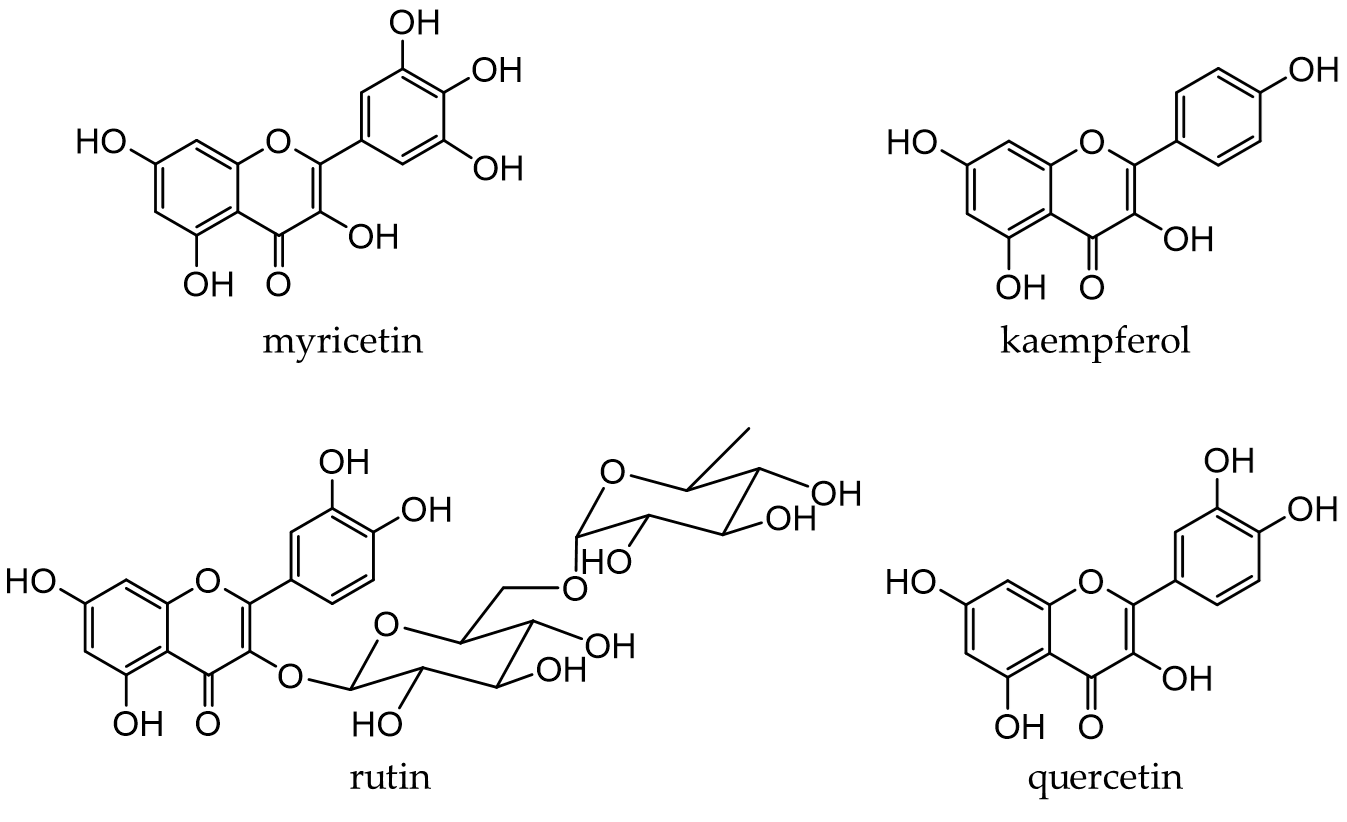
Figure 4. Chemical structures of major flavonols.
4.1. Dietary sources and metabolism of flavonols
4.1.1. Onions
Onions (Allium cepa L.) are used as an ingredient in various dishes. They are rich in flavanols, the most abundant of which is quercetin [73,74]. Quercetin (an aglycone) is mostly present in the outer skin and quercetin 4'-glucoside and quercetin 3,4'-diglucoside in the bulbs, which are generally edible [75,76]. Figure 5 shows the quercetin catabolites produced by intestinal bacteria and phase II enzymes in the liver. Quercetin derivatives in onions increase their bioavailability through cooking processes, such as baking, frying, and grilling [77].
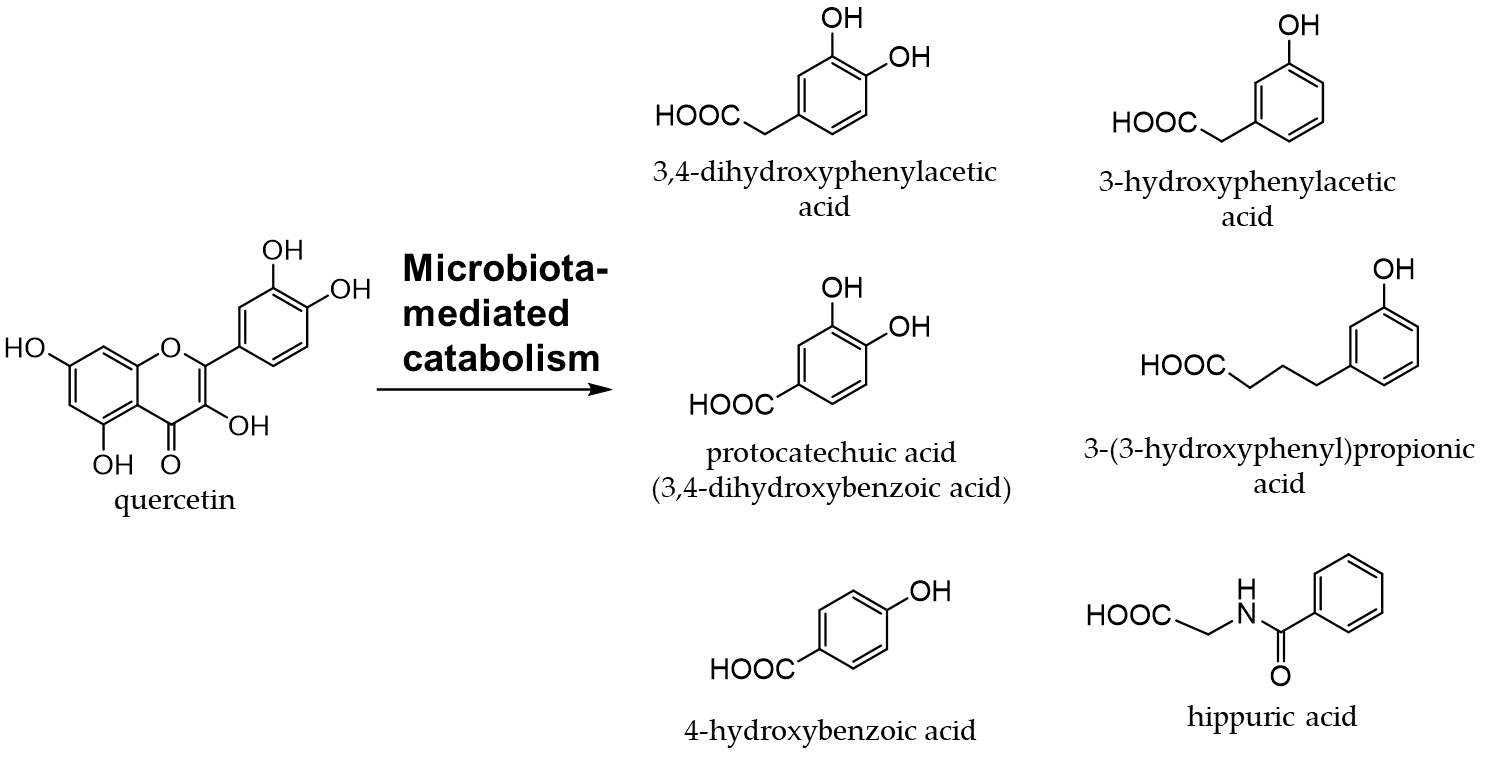
Figure 5. Quercetin catabolites by intestinal bacteria.
4.1.2. Buckwheat
Buckwheat is widely grown in Asia, Europe, and the Americas. Both common buckwheat (Fagopyrum esculentum Moench) and tartary buckwheat (F. tataricum (L.) Gilib.) are used as food sources, and the antioxidant activity of tartary buckwheat is higher than that of common buckwheat [78]. Rutin is the main flavonol in buckwheat, accounting for 90% of all phenolic compounds [79].
4.2. Health benefits of flavonols
4.2.1. Onions
Quercetin exhibits antioxidant, anti-inflammatory, and anti-osteoporotic activities [72,80]. The administration of quercetin and quercetin glycosides extracted from onion skin to rats on a high-fat diet increased serum antioxidant activity and significantly increased enzyme activity derived from intestinal bacteria [81]. In other words, quercetin effectively reduced the intestinal flora abnormalities induced by the high-fat diet. However, in human clinical studies, the administration of onion peel extracts to obese patients with hypertension did not attenuate their symptoms [82]. Similarly, in clinical studies on hypertension and rheumatoid arthritis, the administration of quercetin did not exert beneficial effects [83–87].
Quercetin glycosides are catabolized to produce phenolic acids by intestinal bacteria [88]. Among the phenolic acids derived from quercetin glycosides, 3,4-dihydroxyphenylacetic acid is the most effective at scavenging free radicals and inducing phase II enzymes [89]. Quercetin has been implicated in the attenuation of insulin resistance and atherosclerosis in obesity-related diseases [90–93]. It was found to promote intestinal homeostasis by changing the intestinal flora [94,95] and also plays a role in the prevention and treatment of inflammatory bowel disease [96,97].
4.2.2. Buckwheat
Rutin and quercetin contained in tartary buckwheat regulate gut microbiota and are involved in lipid metabolism [98]. Rutin had little effect on attenuating obesity but tended to decrease fat deposition in the liver [98]. Phenolic compounds extracted from tartary buckwheat bran showed dose-dependent anticancer activity against human breast cancer MDA-MB-231 cells [99].
5. Isoflavones
Isoflavones are flavonoids with 3-phenylchromone as the basic skeleton (Figure 6). They are abundant in plants of the legume family (Fabaceae), such as soybeans and kudzu. Isoflavones bind to estrogen receptors in the body and exert a number of effects because their chemical structures are similar to estrogen [100]. They may be beneficial, but also detrimental [101]. For example, while isoflavones are expected to effectively prevent osteoporosis, breast cancer, and prostate cancer, they also increase the risk of the onset and recurrence of breast cancer [101]. Glycosides are not easily absorbed in the small intestine and must be converted into aglycones, such as genistein and daidzein, to function in vivo [102,103].
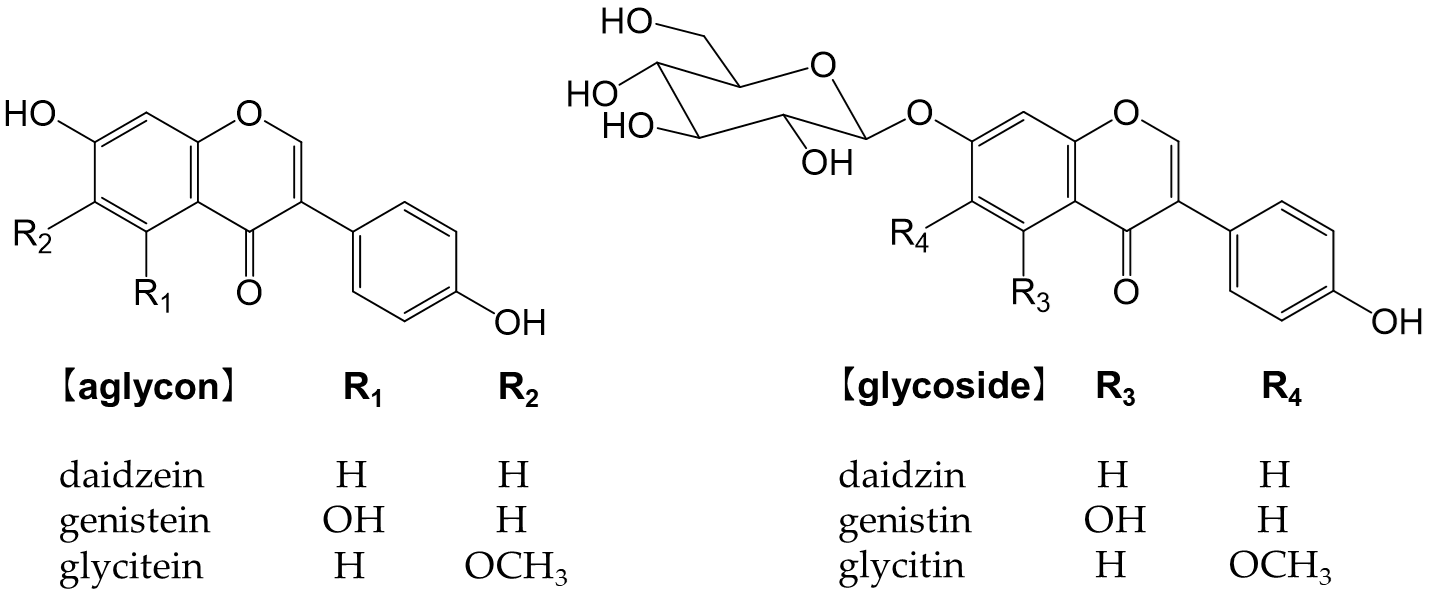
Figure 6. Chemical structure of the main isoflavones and isoflavone glycosides.
Soybeans (Glycine max (L.) Merr.) are the most abundant source of isoflavones [104]. Many isoflavones, such as genistin and daidzin, are present in food (Figure 6). In the small intestine, lactase-phlorizin hydrolase and cytosolic β-glucosidase hydrolyze monoglucuronides to form aglycones [105,106]. The absorbed isoflavone aglycones are mainly metabolized to glucuronides and sulfates by endogenous phase I and phase II enzymes. Isoflavones are excreted into the intestines via the enterohepatic circulation, and unabsorbed isoflavones reach the colon and are metabolized to form the metabolite, equol, and other metabolites by intestinal bacteria [107].
Soybeans are rich in isoflavones, particularly genistin and daidzin [104]. Isoflavones are phytoestrogens, such as the female hormone 17-β-estradiol, which are less active than hormones, but exhibit estrogenic activity [108]. Therefore, the intake of isoflavones is expected to alleviate menopausal symptoms in women, increase bone formation, and reduce the incidence of cardiovascular disease. Equol is a metabolite of daidzin/daidzein formed by intestinal bacteria. It is more stable and more easily absorbed than daidzein [109] and exhibits stronger estrogenic activity than other isoflavones and isoflavone-derived metabolites [110–113]. Isoflavone aglycones and glycosides are both catabolized by enzymes of the intestinal microbiota to produce high levels of antioxidant substances, such as equol. A correlation has been reported between soybean intake and the attenuation of menopausal symptoms [114].
The intake of soy isoflavones has been suggested to reduce bone resorption, prevent some types of cancers, and improve learning [115–118]. These health effects are attributed to equol produced from soy isoflavones by the action of the intestinal microbiota.
6. Phenylpropanoids
Phenylpropanoids, also called lignoids, are compounds that have a C6-C3 skeleton, which consist with a C3 group attached to an aromatic ring. Monomers include caffeic acid, which is widely present in plants, and chlorogenic acid (an ester of caffeic and quinic acid), which is abundant in green coffee beans. Sesamin is a dimer,
also known as lignan, and is abundant in sesame seeds. Chlorogenic acid may be ingested from food. Figure 7 shows the chemical structures of the major phenylpropanoids.
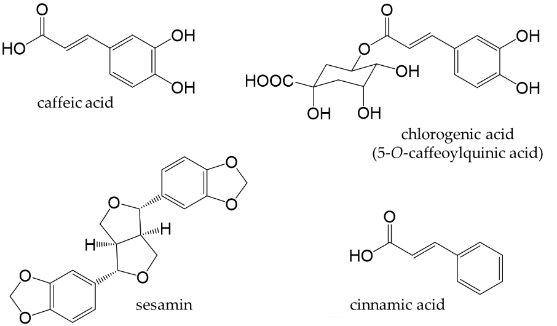
Figure 7. Chemical structures of major phenylpropanoids.
6.1. Dietary source and metabolism of phenylpropanoids
6.1.1. Coffee
Coffee is one of the most consumed beverages in the world. It contains at least 30 types of chlorogenic acids [119]. The term “chlorogenic acids” refers to a group of phenolic compounds, of which approximately 400 have been discovered to date [120]. 5-O-caffeoylquinic acid is the main chlorogenic acid found in green coffee beans. Although the type and concentration of chlorogenic acids vary depending on the type of coffee bean,
the roasting process, and extraction method, the beneficial health effects of coffee are related to its chlorogenic acid content, whether green or roasted. The high antioxidant activity of coffee is attributed to the amount of chlorogenic acid present [121].
6.1.2. Sesame
Sesame (Sesamum indicum L.) is an edible seed and source of high-quality edible oil. Sesame oil exhibits antioxidant activity and possesses health-promoting properties because it contains vitamin E and lignans [122,123]. The major lignans in sesame are sesamin and sesamolin, which are formed by the dimerization of two phenylpropanoids [124].
6.2. Health benefits of phenylpropanoids
6.2.1. Coffee
Chlorogenic acids exhibit antioxidant activity [125–127] and anti-obesity activity in vivo [128–130]. Daily coffee consumption reduces the risk of type 2 diabetes [131]. Chlorogenic acid from coffee possesses prebiotic properties in vivo [132,133]. Therefore, the daily consumption of coffee may contribute to the prevention of obesity and lifestyle-related diseases.
6.2.2. Sesame
The lignans in sesame have a number of health benefits, including anticancer activity, reducing the risk of cardiovascular diseases, and anti-inflammatory effects [134,135]. They are converted into enterolignans by intestinal bacteria and exert their effects as phytoestrogens [136]. Sesame lignans have been shown to inhibit L-tryptophan indole-lyase (TIL) produced by intestinal bacteria and suppress the production of indoxyl sulfate, a uremic toxin, catalyzed by TIL [137]. The inhibition of TIL by sesame lignans has potential as a strategy to prevent and treat chronic kidney diseases. Although sesaminol triglucoside, a sesame lignan glycoside, did not inhibit TIL, it induced significant increases in Lactobacillus and Bifidobacterium and changed the intestinal microbial environment [138].
7. Stilbenoids
Stilbenoids are derivatives of stilbene, an aromatic hydrocarbon called 1,2-diphenylethene. Major stilbenoids are shown in Figure 8. Resveratrol is a type of stilbenoid that is present in many plant food materials, such as grapes, cranberries, red currants, and peanut skin, as well as in their processed products [139]. As a stilbenoid phenolic compound, resveratrol has been extensively studied.
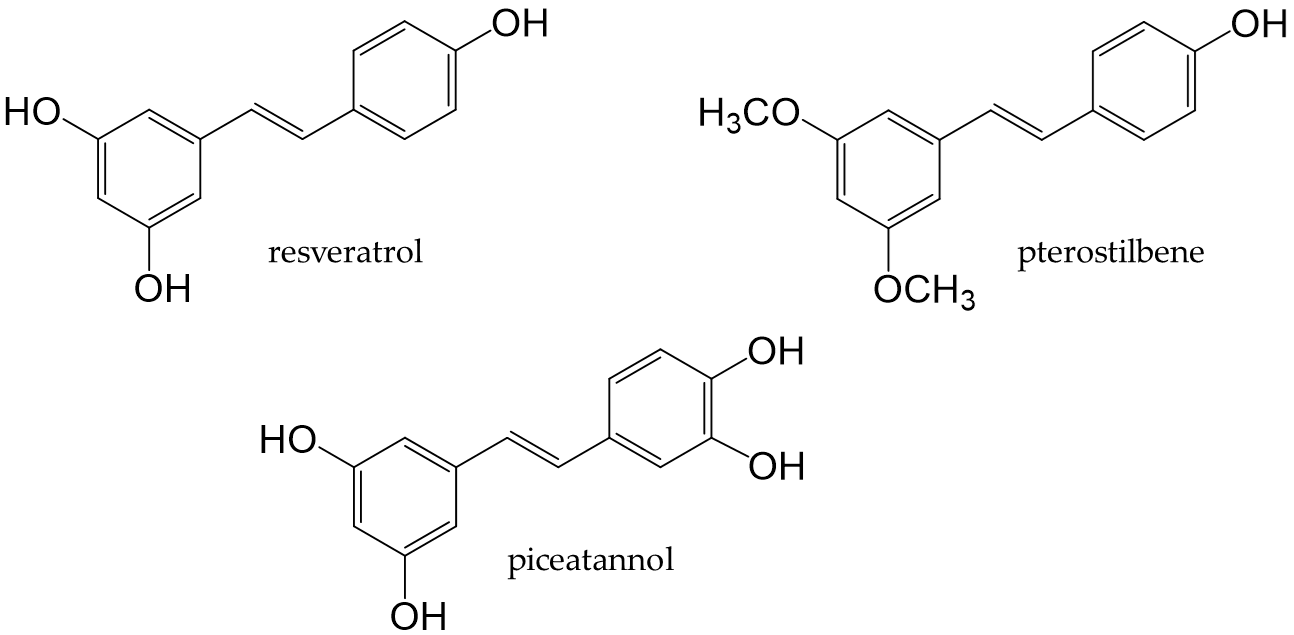
Figure 8. Chemical structures of major stilbenoids.
Resveratrol, a stilbenoid found in many plants, possesses antifungal and antibacterial properties. The food sources that contain resveratrol are grapes, wine [139], and grape seed oil [140]. Resveratrol, in its native state, is present at low amounts in humans, with only 1-8% being detected in serum. Although 75% is absorbed, it is rapidly metabolized [141,142]. Resveratrol undergoes glucuronidation and sulfation in the liver and duodenum to form resveratrol-3-glucuronide (R3G) and resveratrol-3-sulfate (R3S), respectively [143,144].
Resveratrol has been shown to modulate and promote intestinal barrier function in mice, suggesting its potential to augment the intestinal flora [145,146]. Resveratrol prevented obesity and attenuated NAFLD and NASH by modulating the intestinal flora, maintaining intestinal barrier integrity, and suppressing intestinal inflammation in animal models [147–150]. Furthermore, the administration of resveratrol reportedly affected the intestinal flora and steroid metabolism in middle-aged men with metabolic syndrome [143,151–153]; however, the underlying mechanisms have not yet been elucidated. Red wine consumption reduced the risk of coronary heart disease and prevented obesity through the beneficial effects of phenolic compounds in red wine, particularly resveratrol [154,155].
8. Curcuminoids
Turmeric is a spice prepared from the underground stems of Curcuma longa L. It contains curcuminoids, such as curcumin, demethoxycurcumin, and bisdemethoxy-curcumin (Figure 9). Curcumin is the most abundant curcuminoid in turmeric [156] and contains phenolic hydroxyl groups in its chemical structure; therefore, it functions as apotent antioxidant that suppresses the production of ROS [157].
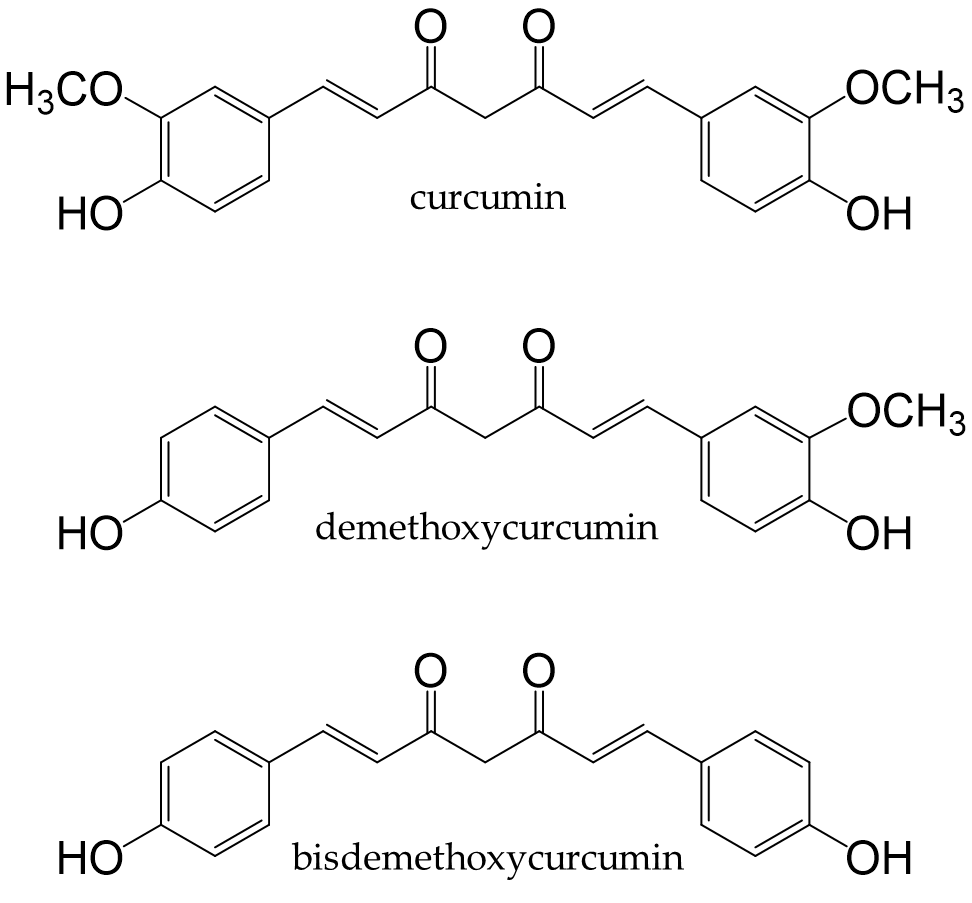
Figure 9. Chemical structures of curcuminoids.
Curcumin exhibits anti-inflammatory, antibacterial, and anti-tumor activities [158–162] and interferes with cancer-associated signaling pathways by targeting proteins and modulating gene expression [163,164]. In human clinical trials, the administration of curcumin capsules to patients with colorectal cancer reduced inflammation and oxidative stress in malignant colorectal epithelial cells. It also attenuated inflammation in patients with UC and gastrointestinal disorders [165–168].
9. Conclusion
Target phenolic compounds must be absorbed to exert their effects, and this requires the cleavage of the sugar of a glycoside. The glycoside is then converted into an aglycone that is subsequently metabolized by phase I and phase II enzymes in the small intestine and liver before circulating in the body. Unabsorbed phenolic compounds undergo biotransformation by intestinal bacteria, after which they are absorbed and circulated in the body. These metabolites exert antioxidant and anti-inflammatory effects.
Although phenolic compounds have been extensively examined in animal and cell culture studies in the last decade, the number of human clinical trials has been insufficient. Research on their effects in humans requires a great deal of effort because detailed planning and massive data collection are required due to large individual differences. Dietary ingredients are safe for consumption, but do not exert immediate effects. Further research on the nutrients present in the daily diet and their beneficial effects is warranted and may provide insights into the prevention or attenuation of diseases.
Author Contributions: Conceptualization, T.I. and Y.M.; Methodology, Y.M. and S.K..; Validation, Y.M. and T.I.; Investigation, Y.M.; Resources, Y.M., M.K., and T.I.; data curation, Y.M.; writing—original draft preparation, Y.M.; writing—review and editing, S.K. and T.I.; visualization, Y.M.; supervision, M.K. and T.I.; Project administration, T.I.; funding acquisition, T.I. All authors have read and agreed to the published version of the manuscript.
Funding: This review was supported by grant obtainted for JSPS KAKENHI, Grant Number JP21K11587.
Institutional Review Board Statement: Animal experiments, cell culture experiments, and clinical trials conducted in the cited studies were approved by the Ethics Committee of each institution and published in journals or books.
Conflicts of Interest: The authors declare no conflicts of interest.
References
- Glevitzky, I.; Dumitrel, G.A.; Glevitzky, M.; Pasca, B.; Otrisal, P.; Bungau, S.; Cioca, G.; Pantis, C.; Popa, M. Statistical Analysis of the Relationship Between Antioxidant Activity and the Structure of Flavonoid Compounds. Rev. Chim. 2019, 70, 3103–3107. https://doi.org/10.37358/RC.19.9.7497.
- Selma, M.V.; Espín, J.C.; Tomás-Barberán, F.A. Interaction between Phenolics and Gut Microbiota: Role in Human Health. J. Agric. Food Chem. 2009, 57, 6485–6501. https://doi.org/10.1021/jf902107d.
- Uddin, M.S.; Kabir, M.T.; Tewari, D.; Al Mamun, A.; Barreto, G.E.; Bungau, S.G.; Bin-Jumah, M.N.; Abdel-Daim, M.M.; Ashraf, G.M. Emerging Therapeutic Promise of Ketogenic Diet to Attenuate Neuropathological Alterations in Alzheimer’s Disease. Mol. Neurobiol. 2020, 57, 4961–4977. https://doi.org/10.1007/s12035-020-02065-3/Published.
- Kumar, S.; Behl, T.; Sachdeva, M.; Sehgal, A.; Kumari, S.; Kumar, A.; Kaur, G.; Yadav, H.N.; Bungau, S. Implicating the Effect of Ketogenic Diet as a Preventive Measure to Obesity and Diabetes Mellitus. Life Sci. 2021, 264, 118661. https://doi.org/10.1016/j.lfs.2020.118661.
- Behl, T.; Bungau, S.; Kumar, K.; Zengin, G.; Khan, F.; Kumar, A.; Kaur, R.; Venkatachalam, T.; Tit, D.M.; Vesa, C.M.; et al. Pleotropic Effects of Polyphenols in Cardiovascular System. Biomed. Pharmacother. 2020, 130, 110714. https://doi.org/10.1016/j.biopha.2020.110714.
- Behl, T.; Upadhyay, T.; Singh, S.; Chigurupati, S.; Alsubayiel, A.M.; Mani, V.; Vargas-De-la-cruz, C.; Uivarosan, D.; Bustea, C.; Sava, C.; et al. Polyphenols Targeting MAPK Mediated Oxidative Stress and Inflammation in Rheumatoid Arthritis. Molecules 2021, 26, 6570. https://doi.org/10.3390/molecules26216570.
- Behl, T.; Mehta, K.; Sehgal, A.; Singh, S.; Sharma, N.; Ahmadi, A.; Arora, S.; Bungau, S. Exploring the Role of Polyphenols in Rheumatoid Arthritis. Crit. Rev. Food Sci. Nutr. 2022, 62, 5372–5393. https://doi.org/10.1080/10408398.2021.1924613.
- Kabra, A.; Garg, R.; Brimson, J.; Živković, J.; Almawash, S.; Ayaz, M.; Nawaz, A.; Hassan, S.S.U.; Bungau, S. Mechanistic Insights into the Role of Plant Polyphenols and Their Nano-Formulations in the Management of Depression. Front. Pharm. 2022, 13, 1046599. https://doi.org/10.3389/fphar.2022.1046599.
- Behl, T.; Rana, T.; Alotaibi, G.H.; Shamsuzzaman, M.; Naqvi, M.; Sehgal, A.; Singh, S.; Sharma, N.; Almoshari, Y.; Abdellatif, A.A.H.; et al. Polyphenols Inhibiting MAPK Signalling Pathway Mediated Oxidative Stress and Inflammation in Depression. Biomed. Pharmacother. 2022, 146, 112545. https://doi.org/10.1016/j.biopha.2021.112545.
- Bungau, S.; Abdel-Daim, M.M.; Tit, D.M.; Ghanem, E.; Sato, S.; Maruyama-Inoue, M.; Yamane, S.; Kadonosono, K. Health Benefits of Polyphenols and Carotenoids in Age-Related Eye Diseases. Oxid. Med. Cell Longev. 2019, 2019, 9783429. https://doi.org/10.1155/2019/9783429.
- Bors, W.; Heller, W.; Michel, C.; Saran, M. Flavonoids as Antioxidants: Determination of Radical-Scavenging Efficiencies. Methods Enzymol. 1990, 186, 343–355.
- Nanjo, F.; Honda, M.; Okushio, K.; Matsumoto, N.; Ishigaki, F.; Ishigami, T.; Hara, Y. Effects of Dietary Tea Catechins on Alpha-Tocopherol Levels, Lipid Peroxidation, and Erythrocyte Deformability in Rats Fed on High Palm Oil and Perilla Oil Diets. Biol. Pharm. Bull. 1993, 16, 1156–1159. https://doi.org/10.1248/bpb.16.1156.
- Manach, C.; Scalbert, A.; Morand, C.; Rémésy, C.; Jiménez, L. Polyphenols: Food Sources and Bioavailability. Am J Clin Nutr 2004, 79, 727–774. https://doi.org/10.1093/ajcn/79.5.727.
- Donovan, J.L.; Manach, C.; Faulks, R.M.; Kroon, P.A In Plant Secondary Metabolites: Occurrence, Structure and Role in the Human Diet; Blackwell Pub:Oxford, UK, 2006; ISBN 9781405125093.
- Bernatoniene, J.; Kopustinskiene, D.M. The Role of Catechins in Cellular Responses to Oxidative Stress. Molecules 2018, 23, 965. https://doi.org/10.3390/molecules23040965.
- Youn, H.S.; Lee, J.Y.; Saitoh, S.I.; Miyake, K.; Kang, K.W.; Choi, Y.J.; Hwang, D.H. Suppression of MyD88- and TRIF-Dependent Signaling Pathways of Toll-like Receptor by (-)-Epigallocatechin-3-Gallate, a Polyphenol Component of Green Tea. Biochem. Pharm. 2006, 72, 850–859. https://doi.org/10.1016/j.bcp.2006.06.021.
- Fraga, C.G.; Galleano, M.; Verstraeten, S.V.; Oteiza, P.I. Basic Biochemical Mechanisms behind the Health Benefits of Polyphenols. Mol. Asp. Med. 2010, 31, 435–445. https://doi.org/10.1016/j.mam.2010.09.006.
- Fan, F.Y.; Sang, L.X.; Jiang, M. Catechins and Their Therapeutic Benefits to Inflammatory Bowel Disease. Molecules 2017, 22, 484. https://doi.org/10.3390/molecules22030484.
- Dias, T.R.; Tomás, G.; Teixeira, N.F.; Alves, M.G.; Oliveira, P.F.; Silva, B. M. White Tea (Camellia sinensis (L.)): Antioxidant Properties And Beneficial Health Effects. Int. J. Food Sci. Nutr. Diet. 2013, 2, 19–26. https://doi.org/10.19070/2326-3350-130005.
- Hattori, M.; Kusumoto, I.T.; Namba, T.; Ishigami, T.; Hara, Y. Effect of Tea Polyphenols on Glucan Synthesis by Glucosyltransferase from Streptococcus Mutans. Chem. Pharm. Bull. 1990, 38, 717–720. https://doi.org/10.1248/cpb.38.717.
- Matsuzaki, T.; Hara, Y. Antioxidative Activity of Tea Leaf Catechins. Nippon Nogeikagaku Kaishi 1985, 59, 129–134. https://doi.org/10.1271/nogeikagaku1924.59.129.
- Dias, T.R.; Alves, M.G.; Casal, S.; Oliveira, P.F.; Silva, B.M. Promising Potential of Dietary (Poly)Phenolic Compounds in the Prevention and Treatment of Diabetes Mellitus. Curr. Med. Chem. 2017, 24, 334–354. https://doi.org/10.2174/0929867323666160905.
- Moderno, P.M.; Carvalho, M.; Silva, B.M. Recent Patents on Camellia Sinensis: Source of Health Promoting Compounds. Recent Pat. Food Nutr. Agric. 2009, 1, 182–192.
- Cho, Y.S.; Schiller, N.L.; Kahng, H.Y.; Oh, K.H. Cellular Responses and Proteomic Analysis of Escherichia Coli Exposed to Green Tea Polyphenols. Curr. Microbiol. 2007, 55, 501–506. https://doi.org/10.1007/s00284-007-9021-8.
- Lee, H.C.; Jenner, A.M.; Low, C.S.; Lee, Y.K. Effect of Tea Phenolics and Their Aromatic Fecal Bacterial Metabolites on Intestinal Microbiota. Res. Microbiol. 2006, 157, 876–884. https://doi.org/10.1016/j.resmic.2006.07.004.
- Pereira-Caro, G.; Moreno-Rojas, J.M.; Brindani, N.; del Rio, D.; Lean, M.E.J.; Hara, Y.; Crozier, A. Bioavailability of Black Tea Theaflavins: Absorption, Metabolism, and Colonic Catabolism. J. Agric. Food Chem. 2017, 65, 5365–5374. https://doi.org/10.1021/acs.jafc.7b01707.
- Liu, Z.; de Bruijn, W.J.C.; Sanders, M.G.; Wang, S.; Bruins, M.E.; Vincken, J.P. Insights in the Recalcitrance of Theasinensin A to Human Gut Microbial Degradation. J. Agric. Food Chem. 2021, 69, 2477–2484. https://doi.org/10.1021/acs.jafc.1c00727.
- Liu, Z.; de Bruijn, W.J.C.; Bruins, M.E.; Vincken, J.P. Microbial Metabolism of Theaflavin-3,3′-Digallate and Its Gut Microbiota Composition Modulatory Effects. J. Agric. Food Chem. 2021, 69, 232–245. https://doi.org/10.1021/acs.jafc.0c06622.
- Mulder, T.P.J.; van Platerink, C.J.; Schuyl, P.J.W.; van Amelsvoort, J.M.M. Analysis of Theaflavins in Biological Fluids Using Liquid Chromatography-Electrospray Mass Spectrometry. J. Chromatogr. B Biomed. Sci. Appl. 2001, 760, 271–279. https://doi.org/10.1016/s0378-4347(01)00285-7.
- Hashimoto, F.; Nonaka, G.; Nishioka, I. Tannins and Related Compounds. LXIX. : Isolation and Structure Elucidation of B, B’-Linked Bisflavanoids, Theasinensins D-G and Oolongtheanin from Oolong Tea. (2). Chem. Pharm. Bull. 1988, 36, 1676–1684. https://doi.org/10.1248/cpb.36.1676.
- Sorrenti, V.; Ali, S.; Mancin, L.; Davinelli, S.; Paoli, A.; Scapagnini, G. Cocoa Polyphenols and Gut Microbiota Interplay: Bioavailability, Prebiotic Effect, and Impact on Human Health. Nutrients 2020, 12, 1908. https://doi.org/10.3390/nu12071908.
- Flores, M.E.J. Cocoa Flavanols: Natural Agents with Attenuating Effects on Metabolic Syndrome Risk Factors. Nutrients 2019, 11, 751. https://doi.org/10.3390/nu11040751.
- Natsume, M.; Osakabe, N.; Yamagishi, M.; Takizawa, T.; Nakamura, T.; Miyatake, H.; Hatano, T.; Yoshida, T. Analyses of Polyphenols in Cacao Liquor, Cocoa, and Chocolate by Normal-Phase and Reversed-Phase HPLC. Biosci. Biotechnol. Biochem. 2000, 64, 2581–2587. https://doi.org/10.1271/bbb.64.2581.
- Borchers, A.T.; Keen, C.L.; Hannum, S.M.; Gershwin, M.E. Cocoa and Chocolate: Composition, Bioavailability, and Health Implications. J. Med. Food 2000, 3, 77–105. https://doi.org/10.1089/109662000416285.
- Maldonado-Mateus, L.Y.; Perez-Burillo, S.; Lerma-Aguilera, A.; Hinojosa-Nogueira, D.; Ruíz-Pérez, S.; Gosalbes, M.J.; Francino, M.P.; Rufián-Henares, J.Á.; Pastoriza De La Cueva, S. Effect of Roasting Conditions on Cocoa Bioactivity and Gut Microbiota Modulation. Food Funct. 2021, 12, 9680–9692. https://doi.org/10.1039/d1fo01155c.
- Gómez-Juaristi, M.; Sarria, B.; Martínez-López, S.; Clemente, L.B.; Mateos, R. Flavanol Bioavailability in Two Cocoa Products with Different Phenolic Content. A Comparative Study in Humans. Nutrients 2019, 11, 1441. https://doi.org/10.3390/nu11071441.
- Loke, W.M.; Hodgson, J.M.; Proudfoot, J.M.; Mckinley, A.J.; Puddey, I.B.; Croft, K.D. Pure Dietary Flavonoids Quercetin and (-)-Epicatechin Augment Nitric Oxide Products and Reduce Endothelin-1 Acutely in Healthy Men. Am. J. Clin. Nutr. 2008, 88, 1018–1025. https://doi.org/10.1093/ajcn/88.4.1018.
- Schroeter, H.; Heiss, C.; Balzer, J.; Kleinbongard, P.; Keen, C.L.; Hollenberg, N.K.; Sies, H.; Kwik-Uribe, C.; Schmitz, H.H.; Kelm, M. (-)-Epicatechin Mediates Beneficial Effects of Flavanol-Rich Cocoa on Vascular Function in Humans. Proc. Natl. Acad. Sci. USA 2006, 103, 1024–1029. https://doi.org/10.1073/pnas.0510168103.
- Ottaviani, J.I.; Borges, G.; Momma, T.Y.; Spencer, J.P.E.; Keen, C.L.; Crozier, A.; Schroeter, H. The Metabolome of [2-14C](-)-Epicatechin in Humans: Implications for the Assessment of Efficacy, Safety, and Mechanisms of Action of Polyphenolic Bioactives. Sci. Rep. 2016, 6, 29034. https://doi.org/10.1038/srep29034.
- Cremonini, E.; Wang, Z.; Bettaieb, A.; Adamo, A.M.; Daveri, E.; Mills, D.A.; Kalanetra, K.M.; Haj, F.G.; Karakas, S.; Oteiza, P.I. (-)-Epicatechin Protects the Intestinal Barrier from High Fat Diet-Induced Permeabilization: Implications for Steatosis and Insulin Resistance. Redox Biol. 2018, 14, 588–599. https://doi.org/10.1016/j.redox.2017.11.002.
- Corral-Jara, K.F.; Nuthikattu, S.; Rutledge, J.; Villablanca, A.; Fong, R.; Heiss, C.; Ottaviani, J.I.; Milenkovic, D. Structurally Related (-)-Epicatechin Metabolites and Gut Microbiota Derived Metabolites Exert Genomic Modifications via VEGF Signaling Pathways in Brain Microvascular Endothelial Cells under Lipotoxic Conditions: Integrated Multi-Omic Study. J. Proteom. 2022, 263, 104603. https://doi.org/10.1016/j.jprot.2022.104603.
- Li, B.Y.; Li, H.Y.; Zhou, D.D.; Huang, S.Y.; Luo, M.; Gan, R.Y.; Mao, Q.Q.; Saimaiti, A.; Shang, A.; Li, H. bin Effects of Different Green Tea Extracts on Chronic Alcohol Induced-Fatty Liver Disease by Ameliorating Oxidative Stress and Inflammation in Mice. Oxid. Med. Cell Longev. 2021, 2021, 5188205. https://doi.org/10.1155/2021/5188205.
- Zhao, L.; Wang, S.; Zhang, N.; Zhou, J.; Mehmood, A.; Raka, R.N.; Zhou, F.; Zhao, L. The Beneficial Effects of Natural Extracts and Bioactive Compounds on the Gut-Liver Axis: A Promising Intervention for Alcoholic Liver Disease. Antioxidants 2022, 11, 1211. https://doi.org/10.3390/antiox11061211.
- Li, B.; Mao, Q.; Zhou, D.; Luo, M.; Gan, R.; Li, H.; Huang, S.; Saimaiti, A.; Shang, A.; Li, H. Effects of Tea against Alcoholic Fatty Liver Disease by Modulating Gut Microbiota in Chronic Alcohol-Exposed Mice. Foods 2021, 10, 1232. https://doi.org/10.3390/foods10061232.
- Naito, Y.; Ushiroda, C.; Mizushima, K.; Inoue, R.; Yasukawa, Z.; Abe, A.; Takagi, T.; Gastroenterology, M. Epigallocatechin-3-Gallate (EGCG) Attenuates Non-Alcoholic Fatty Liver Disease via Modulating the Interaction between Gut Microbiota and Bile Acids. J. Clin. Biochem. Nutr 2020, 67, 2–9. https://doi.org/10.3164/jcbn.20039.
- Sharma, S.P.; Suk, K.T.; Kim, D.J. Significance of Gut Microbiota in Alcoholic and Non-Alcoholic Fatty Liver Diseases. World J. Gastroenterol. 2021, 27, 6161–6179. https://doi.org/10.3748/wjg.v27.i37.6161.
- He, L.H.; Yao, D.H.; Wang, L.Y.; Zhang, L.; Bai, X.L. Gut Microbiome-Mediated Alteration of Immunity, Inflammation, and Metabolism Involved in the Regulation of Non-Alcoholic Fatty Liver Disease. Front. Microbiol. 2021, 12, 761836. https://doi.org/10.3389/fmicb.2021.761836.
- Zhou, K. Strategies to Promote Abundance of Akkermansia Muciniphila, an Emerging Probiotics in the Gut, Evidence from Dietary Intervention Studies. J. Funct. Foods 2017, 33, 194–201. https://doi.org/10.1016/j.jff.2017.03.045.
- Roopchand, D.E.; Carmody, R.N.; Kuhn, P.; Moskal, K.; Rojas-Silva, P.; Turnbaugh, P.J.; Raskin, I. Dietary Polyphenols Promote Growth of the Gut Bacterium Akkermansia Muciniphila and Attenuate High-Fat Diet-Induced Metabolic Syndrome. Diabetes 2015, 64, 2847–2858. https://doi.org/10.2337/db14-1916.
- Dryden, G.W.; Lam, A.; Beatty, K.; Qazzaz, H.H.; McClain, C.J. A Pilot Study to Evaluate the Safety and Efficacy of an Oral Dose of (−)-Epigallocatechin-3-Gallate–Rich Polyphenon E in Patients With Mild to Moderate Ulcerative Colitis. Inflamm. Bowel. Dis. 2013, 19, 1904–1912. https://doi.org/10.1097/MIB.0b013e31828f5198.
- Vasconcelos, P.C.D.P.; Seito, L.N.; di Stasi, L.C.; Akiko Hiruma-Lima, C.; Pellizzon, C.H. Epicatechin Used in the Treatment of Intestinal Inflammatory Disease: An Analysis by Experimental Models. Evid.-Based Complement. Altern. Med. 2012, 2012, 508902. https://doi.org/10.1155/2012/508902.
- Brückner, M.; Westphal, S.; Domschke, W.; Kucharzik, T.; Lügering, A. Green Tea Polyphenol Epigallocatechin-3-Gallate Shows Therapeutic Antioxidative Effects in a Murine Model of Colitis. J. Crohns Colitis 2012, 6, 226–235. https://doi.org/10.1016/j.crohns.2011.08.012.
- Rodríguez-Ramiro, I.; Martín, M.Á.; Ramos, S.; Bravo, L.; Goya, L. Comparative Effects of Dietary Flavanols on Antioxidant Defenses and Their Response to Oxidant-Induced Stress on Caco2 Cells. Eur. J. Nutr. 2011, 50, 313–322. https://doi.org/10.1007/s00394-010-0139-2.
- Cecarini, V.; Cuccioloni, M.; Zheng, Y.; Bonfili, L.; Gong, C.; Angeletti, M.; Mena, P.; del Rio, D.; Eleuteri, A.M. Flavan-3-Ol Microbial Metabolites Modulate Proteolysis in Neuronal Cells Reducing Amyloid-Beta (1-42) Levels. Mol. Nutr. Food Res. 2021, 65, 2100380. https://doi.org/10.1002/mnfr.202100380.
- Bao, J.; Liu, W.; Zhou, H.Y.; Gui, Y.R.; Yang, Y.H.; Wu, M.J.; Xiao, Y.F.; Shang, J.T.; Long, G.F.; Shu, X.J. Epigallocatechin-3-Gallate Alleviates Cognitive Deficits in APP/PS1 Mice. Curr. Med. Sci. 2020, 40, 18–27. https://doi.org/10.1007/s11596-020-2142-z.
- Xu, Q.; Langley, M.; Kanthasamy, A.G.; Reddy, M.B. Epigallocatechin Gallate Has a Neurorescue Effect in a Mouse Model of Parkinson Disease. J. Nutr. 2017, 147, 1926–1931. https://doi.org/10.3945/jn.117.255034.
- Sergi, C.M. Epigallocatechin Gallate for Parkinson’s Disease. Clin. Exp. Pharm. Physiol. 2022, 49, 1029–1041. https://doi.org/10.1111/1440-1681.13691.
- Kim, S.R.; Seong, K.J.; Kim, W.J.; Jung, J.Y. Epigallocatechin Gallate Protects against Hypoxia-Induced Inflammation in Microglia via NF-ΚB Suppression and Nrf-2/HO-1 Activation. Int. J. Mol. Sci. 2022, 23, 4004. https://doi.org/10.3390/ijms23074004.
- Jang, S.; Sun, J.; Chen, P.; Lakshman, S.; Molokin, A.; Harnly, J.M.; Vinyard, B.T.; Urban, J.F.; Davis, C.D.; Solano-Aguilar, G. Flavanol-Enriched Cocoa Powder Alters the Intestinal Microbiota, Tissue and Fluid Metabolite Profiles, and Intestinal Gene Expression in Pigs. J. Nutr. 2016, 146, 673–680. https://doi.org/10.3945/jn.115.222968.
- Tzounis, X.; Rodriguez-Mateos, A.; Vulevic, J.; Gibson, G.R.; Kwik-Uribe, C.; Spencer, J.P.E. Prebiotic Evaluation of Cocoa-Derived Flavanols in Healthy Humans by Using a Randomized, Controlled, Double-Blind, Crossover Intervention Study. Am. J. Clin. Nutr. 2011, 93, 62–72. https://doi.org/10.3945/ajcn.110.000075.
- Pérez-Cano, F.J.; Massot-Cladera, M.; Franch, À.; Castellote, C.; Castell, M. The Effects of Cocoa on the Immune System. Front. Pharm. 2013, 4, 71. https://doi.org/10.3389/fphar.2013.00071.
- Massot-Cladera, M.; Pérez-Berezo, T.; Franch, A.; Castell, M.; Pérez-Cano, F.J. Cocoa Modulatory Effect on Rat Faecal Microbiota and Colonic Crosstalk. Arch. Biochem. Biophys. 2012, 527, 105–112. https://doi.org/10.1016/j.abb.2012.05.015
- Álvarez-Cilleros, D.; Ramos, S.; López-Oliva, M.E.; Escrivá, F.; Álvarez, C.; Fernández-Millán, E.; Martín, M.Á. Cocoa Diet Modulates Gut Microbiota Composition and Improves Intestinal Health in Zucker Diabetic Rats. Food Res. Int. 2020, 132, 109058. https://doi.org/10.1016/j.foodres.2020.109058.
- Rodríguez-Ramiro, I.; Ramos, S.; López-Oliva, E.; Agis-Torres, A.; Bravo, L.; Goya, L.; Martín, M.A. Cocoa Polyphenols Prevent Inflammation in the Colon of Azoxymethane-Treated Rats and in TNF-α-Stimulated Caco-2 Cells. Br. J. Nutr. 2013, 110, 206–215. https://doi.org/10.1017/S0007114512004862.
- Agostoni, C.; Bresson, J.-L.; Fairweather-Tait, S.; Flynn, A.; Golly, I.; Korhonen, H.; Lagiou, P.; Løvik, M.; Marchelli, R.; Martin, A.; et al. Scientific Opinion on the Substantiation of a Health Claim Related to Cocoa Flavanols and Maintenance of Normal Endothelium‐dependent Vasodilation Pursuant to Article 13(5) of Regulation (EC) No 1924/2006. EFSA J. 2012, 10, 2809. https://doi.org/10.2903/j.efsa.2012.2809.
- Sesso, H.D.; Manson, J.E.; Aragaki, A.K.; Rist, P.M.; Johnson, L.G.; Friedenberg, G.; Copeland, T.; Clar, A.; Mora, S.; Moorthy, M.V.; et al. Effect of Cocoa Flavanol Supplementation for the Prevention of Cardiovascular Disease Events: The COcoa Supplement and Multivitamin Outcomes Study (COSMOS) Randomized Clinical Trial. Am. J. Clin. Nutr. 2022, 115, 1490–1500. https://doi.org/10.1093/ajcn/nqac055.
- Zhu, W.; Lin, K.; Li, K.; Deng, X.; Li, C. Reshaped Fecal Gut Microbiota Composition by the Intake of High Molecular Weight Persimmon Tannin in Normal and High-Cholesterol Diet-Fed Rats. Food Funct. 2018, 9, 541–551. https://doi.org/10.1039/c7fo00995j.
- Matsumura, Y.; Kitabatake, M.; Ouji-Sageshima, N.; Yasui, S.; Mochida, N.; Nakano, R.; Kasahara, K.; Tomoda, K.; Yano, H.; Kayano, S.-i.; et al. Persimmon-Derived Tannin Has Bacteriostatic and Anti-Inflammatory Activity in a Murine Model of Mycobacterium Avium Complex (MAC) Disease. PLoS ONE 2017, 12, e0183489. https://doi.org/10.1371/journal.pone.0183489.
- Matsumura, Y.; Ito, T.; Yano, H.; Kita, E.; Mikasa, K.; Okada, M.; Furutani, A.; Murono, Y.; Shibata, M.; Nishii, Y.; et al. Antioxidant Potential in Non-Extractable Fractions of Dried Persimmon (Diospyros kaki Thunb.). Food Chem. 2016, 202, 99–103. https://doi.org/10.1016/j.foodchem.2016.01.112.
- Kitabatake, M.; Matsumura, Y.; Ouji-Sageshima, N.; Nishioka, T.; Hara, A.; Kayano, S.-i.; Ito, T. Persimmon-Derived Tannin Ameliorates the Pathogenesis of Ulcerative Colitis in a Murine Model through Inhibition of the Inflammatory Response and Alteration of Microbiota. Sci. Rep. 2021, 11, 7286. https://doi.org/10.1038/s41598-021-86608-1.
- Shabbir, U.; Rubab, M.; Daliri, E.B.M.; Chelliah, R.; Javed, A.; Oh, D.H. Curcumin, Quercetin, Catechins and Metabolic Diseases: The Role of Gut Microbiota. Nutrients 2021, 13, 206. https://doi.org/10.3390/nu13010206.
- Murota, K.; Nakamura, Y.; Uehara, M. Flavonoid Metabolism: The Interaction of Metabolites and Gut Microbiota. Biosci. Biotechnol. Biochem. 2018, 82, 600–610. https://doi.org/10.1080/09168451.2018.1444467.
- Slimestad, R.; Fossen, T.; Vågen, I.M. Onions: A Source of Unique Dietary Flavonoids. J. Agric. Food Chem. 2007, 55, 10067–10080. https://doi.org/10.1021/jf0712503.
- Lee, E.J.; Patil, B.S.; Yoo, K.S. Antioxidants of 15 Onions with White, Yellow, and Red Colors and Their Relationship with Pungency, Anthocyanin, and Quercetin. LWT Food Sci. Technol. 2015, 63, 108–114. https://doi.org/10.1016/j.lwt.2015.03.028.
- Benítez, V.; Mollá, E.; Martín-Cabrejas, M.A.; Aguilera, Y.; López-Andréu, F.J.; Cools, K.; Terry, L.A.; Esteban, R.M. Characterization of Industrial Onion Wastes (Allium Cepa, L.): Dietary Fibre and Bioactive Compounds. Plant Foods Hum. Nutr. 2011, 66, 48–57. https://doi.org/10.1007/s11130-011-0212-x.
- Sharma, K.; Asnin, L.; Ko, E.Y.; Lee, E.T.; Park, S.W. Phytochemical Composition of Onion during Long-Term Storage. Acta Agric. Scand B Soil Plant Sci. 2015, 65, 150–160. https://doi.org/10.1080/09064710.2014.983151.
- Cattivelli, A.; Conte, A.; Martini, S.; Tagliazucchi, D. Influence of Cooking Methods on Onion Phenolic Compounds Bioaccessibility. Foods 2021, 10, 1023. https://doi.org/10.3390/foods10051023.
- Sinkovič, L.; Kokalj Sinkovič, D.; Meglič, V. Milling Fractions Composition of Common (Fagopyrum Esculentum Moench) and Tartary (Fagopyrum tataricum (L.) Gaertn.) Buckwheat. Food Chem. 2021, 365, 130459. https://doi.org/10.1016/j.foodchem.2021.130459.
- Sytar, O.; Biel, W.; Smetanska, I.; Brestic, M. Bioactive Compounds and Their Biofunctional Properties of Different Buckwheat Germplasms for Food Processing. In Buckwheat Germplasm in the World; Academic Press: London, UK, 2018; Chapter 19, pp. 191–204. https://doi.org/10.1016/B978-0-12-811006-5.00019-7
- Formica, J.V; Regelson, W. Review of the Biology of Quercetin and Related Bioflavonoids. Food Chem. Toxic 1995, 33, 1061–1080. https://doi.org/10.1016/0278-6915(95)00077-1.
- Grzelak-Błaszczyk, K.; Milala, J.; Kosmala, M.; Kołodziejczyk, K.; Sójka, M.; Czarnecki, A.; Klewicki, R.; Juśkiewicz, J.; Fotschki, B.; Jurgoński, A. Onion Quercetin Monoglycosides Alter Microbial Activity and Increase Antioxidant Capacity. J. Nutr. Biochem. 2018, 56, 81–88. https://doi.org/10.1016/j.jnutbio.2018.02.002.
- Brüll, V.; Burak, C.; Stoffel-Wagner, B.; Wolffram, S.; Nickenig, G.; Müller, C.; Langguth, P.; Alteheld, B.; Fimmers, R.; Stehle, P.; et al. No Effects of Quercetin from Onion Skin Extract on Serum Leptin and Adiponectin Concentrations in Overweight-to-Obese Patients with (Pre-)Hypertension: A Randomized Double-Blinded, Placebo-Controlled Crossover Trial. Eur. J. Nutr. 2017, 56, 2265–2275. https://doi.org/10.1007/s00394-016-1267-0.
- Dower, J.I.; Geleijnse, J.M.; Gijsbers, L.; Schalkwijk, C.; Kromhout, D.; Hollman, P.C. Supplementation of the Pure Flavonoids Epicatechin and Quercetin Affects Some Biomarkers of Endothelial Dysfunction and Inflammation in (Pre)Hypertensive Adults: A Randomized Double-Blind, Placebo-Controlled, Crossover Trial. J. Nutr. 2015, 145, 1459–1463. https://doi.org/10.3945/jn.115.211888.
- Lee, K.H.; Park, E.; Lee, H.J.; Kim, M.O.; Cha, Y.J.; Kim, J.M.; Lee, H.; Shin, M.J. Effects of Daily Quercetin-Rich Supplementation on Cardiometabolic Risks in Male Smokers. Nutr. Res. Pract. 2011, 5, 28–33. https://doi.org/10.4162/nrp.2011.5.1.28.
- Zahedi, M.; Ghiasvand, R.; Feizi, A.; Asgari, G.; Darvish, L. Does Quercetin Improve Cardiovascular Risk Factors and Inflammatory Biomarkers in Women with Type 2 Diabetes: A Double-Blind Randomized Controlled Clinical Trial. Int. J. Prev. Med. 2013, 4, 777–785.
- Rezvan, N.; Moini, A.; Janani, L.; Mohammad, K.; Saedisomeolia, A.; Nourbakhsh, M.; Gorgani-Firuzjaee, S.; Mazaherioun, M.; Hosseinzadeh-Attar, M.J. Effects of Quercetin on Adiponectin-Mediated Insulin Sensitivity in Polycystic Ovary Syndrome: A Randomized Placebo-Controlled Double-Blind Clinical Trial. Horm. Metab. Res. 2017, 49, 115–121. https://doi.org/10.1055/s-0042-118705.
- Javadi, F.; Eghtesadi, S.; Ahmadzadeh, A.; Aryaeian, N.; Zabihiyeganeh, M.; Foroushani, A.R.; Jazayeri, S. The Effect of Quercetin on Plasma Oxidative Status, C-Reactive Protein and Blood Pressure in Women with Rheumatoid Arthritis. Int. J. Prev. Med. 2014, 5, 293–301.
- Mullen, W.; Rouanet, J.-M.; Auger, C.; Teissèdre, P.-L.; Caldwell, S.T.; Hartley, R.C.; Lean, M.E.J.; Edwards, C.A.; Crozier, A. Bioavailability of [2-14C]Quercetin-4′-Glucoside in Rats. J. Agric. Food Chem. 2008, 56, 12127–12137. https://doi.org/10.1021/jf802754s.
- Tang, Y.; Nakashima, S.; Saiki, S.; Myoi, Y.; Abe, N.; Kuwazuru, S.; Zhu, B.; Ashida, H.; Murata, Y.; Nakamura, Y. 3,4-Dihydroxyphenylacetic Acid Is a Predominant Biologically-Active Catabolite of Quercetin Glycosides. Food Res. Int. 2016, 89, 716–723. https://doi.org/10.1016/j.foodres.2016.09.034.
- Carlsen, I.; Frøkiaer, J.; Nørregaard, R. Quercetin Attenuates Cyclooxygenase-2 Expression in Response to Acute Ureteral Obstruction. Am. J. Physiol. Ren. Physiol. 2015, 308, F1297–F1305. https://doi.org/10.1152/ajprenal.00514.2014.-Unilateral.
- Yang, Y.; Chen, G.; Yang, Q.; Ye, J.; Cai, X.; Tsering, P.; Cheng, X.; Hu, C.; Zhang, S.; Cao, P. Gut Microbiota Drives the Attenuation of Dextran Sulphate Sodium-Induced Colitis by Huangqin Decoction. Oncotarget 2017, 8, 48863–48874.
- Forney, L.A.; Lenard, N.R.; Stewart, L.K.; Henagan, T.M. Dietary Quercetin Attenuates Adipose Tissue Expansion and Inflammation and Alters Adipocyte Morphology in a Tissue-Specific Manner. Int. J. Mol. Sci. 2018, 19, 895. https://doi.org/10.3390/ijms19030895.
- Overman, A.; Chuang, C.C.; McIntosh, M. Quercetin Attenuates Inflammation in Human Macrophages and Adipocytes Exposed to Macrophage-Conditioned Media. Int. J. Obes. 2011, 35, 1165–1172. https://doi.org/10.1038/ijo.2010.272.
- Ju Songwen; Ge Yan; Li Ping; Tian Xinxin; Wang Haiyan; Zheng Xiaocui; Ju Songguang Dietary Quercetin Ameliorates Experimental Colitis in Mouse by Remodeling the Function of Colonic Macrophages via a Heme Oxygenase-1-Dependent Pathway. Cell Cycle 2018, 17, 53–63. https://doi.org/10.1080/15384101.2017.1387701.
- Shi, T.; Bian, X.; Yao, Z.; Wang, Y.; Gao, W.; Guo, C. Quercetin Improves Gut Dysbiosis in Antibiotic-Treated Mice. Food Funct. 2020, 11, 8003–8013. https://doi.org/10.1039/d0fo01439g.
- Lin, R.; Piao, M.; Song, Y. Dietary Quercetin Increases Colonic Microbial Diversity and Attenuates Colitis Severity in Citrobacter Rodentium-Infected Mice. Front. Microbiol. 2019, 10, 1092. https://doi.org/10.3389/fmicb.2019.01092.
- Sato, S.; Mukai, Y. Modulation of Chronic Inflammation by Quercetin: The Beneficial Effects on Obesity. J. Inflamm. Res. 2020, 13, 421–431. https://doi.org/10.2147/JIR.S228361.
- Peng, L.; Zhang, Q.; Zhang, Y.; Yao, Z.; Song, P.; Wei, L.; Zhao, G.; Yan, Z. Effect of Tartary Buckwheat, Rutin, and Quercetin on Lipid Metabolism in Rats during High Dietary Fat Intake. Food Sci. Nutr. 2020, 8, 199–213. https://doi.org/10.1002/fsn3.1291.
- Li, F.; Zhang, X.; Li, Y.; Lu, K.; Yin, R.; Ming, J. Phenolics Extracted from Tartary (Fagopyrum tartaricum, L. Gaerth) Buckwheat Bran Exhibit Antioxidant Activity, and an Antiproliferative Effect on Human Breast Cancer MDA-MB-231 Cells through the P38/MAP Kinase Pathway. Food Funct. 2017, 8, 177–188. https://doi.org/10.1039/C6FO01230B.
- Křížová, L.; Dadáková, K.; Kašparovská, J.; Kašparovský, T. Isoflavones. Molecules 2019, 24, 1076. https://doi.org/10.3390/molecules24061076.
- Kim, I.-S. Current Perspectives on the Beneficial Effects of Soybean Isoflavones and Their Metabolites for Humans. Antioxidants 2021, 10, 1064. https://doi.org/10.3390/antiox10071064.
- Shinkaruk, S.; Carreau, C.; Flouriot, G.; Bennetau-Pelissero, C.; Potier, M. Comparative Effects of R- and S-Equol and Implication of Transactivation Functions (AF-1 and AF-2) in Estrogen Receptor-Induced Transcriptional Activity. Nutrients 2010, 2, 340–354. https://doi.org/10.3390/nu2030340.
- Setchell, K.D.R.; Brown, N.M.; Desai, P.; Zimmer-Nechemias, L.; Wolfe, B.E.; Brashear, W.T.; Kirschner, A.S.; Cassidy, A.; Heubi, J.E. Bioavailability of Pure Isoflavones in Healthy Humans and Analysis of Commercial Soy Isoflavone Supplements. J. Nutr. 2001, 131, 1362S-1375S. https://doi.org/10.1093/jn/131.4.1362S.
- Aguiar, C.L.; Baptista, A.S.; Alencar, S.M.; Haddad, R.; Eberlin, M.N. Analysis of Isoflavonoids from Leguminous Plant Extracts by RPHPLC/DAD and Electrospray Ionization Mass Spectrometry. Int. J. Food Sci. Nutr. 2007, 58, 116–124. https://doi.org/10.1080/09637480601149350.
- Day, A.J.; DuPont, M.S.; Ridley, S.; Rhodes, M.; Rhodes, M.J.C.; Morgan, M.R.A.; Williamson, G. Deglycosylation of Flavonoid and Isoflavonoid Glycosides by Human Small Intestine and Liver β-Glucosidase Activity. FEBS Lett. 1998, 436, 71–75. https://doi.org/10.1016/S0014-5793(98)01101-6.
- Day, A.J.; Cañada, F.J.; Dı́az, J.C.; Kroon, P.A.; Mclauchlan, R.; Faulds, C.B.; Plumb, G.W.; Morgan, M.R.A.; Williamson, G. Dietary Flavonoid and Isoflavone Glycosides Are Hydrolysed by the Lactase Site of Lactase Phlorizin Hydrolase. FEBS Lett. 2000, 468, 166–170. https://doi.org/10.1016/S0014-5793(00)01211-4.
- Mayo, B.; Vázquez, L.; Flórez, A.B. Equol: A Bacterial Metabolite from the Daidzein Isoflavone and Its Presumed Beneficial Health Effects. Nutrients 2019, 11, 2231. https://doi.org/10.3390/nu11092231.
- Vitale, D.C.; Piazza, C.; Melilli, B.; Drago, F.; Salomone, S. Isoflavones: Estrogenic Activity, Biological Effect and Bioavailability. Eur. J. Drug Metab. Pharm. 2013, 38, 15–25. https://doi.org/10.1007/s13318-012-0112-y.
- Setchell, D.R.K.; Faughnan, M.S.; Avades, T.; Zimmer-Nechemias, L.; Brown, N.M.; Wolfe, B.E.; Brashear, W.T.; Desai, P.; Oldfield, M.F.; Botting, N.P.; et al. Comparing the Pharmacokinetics of Daidzein and Genistein with the Use of 13 C-Labeled Tracers in Premenopausal Women. Am. J. Clin. Nutr. 2003, 77, 411–419. https://doi.org/10.1093/ajcn/77.2.411 Abstract.
- Setchell, K.D.; Clerici, C.; Lephart, E.D.; Cole, S.J.; Heenan, C.; Castellani, D.; Wolfe, B.E.; Nechemias-Zimmer, L.; Brown, N.M.; Lund, T.D.; et al. S-Equol, a Potent Ligand for Estrogen Receptor β, Is the Exclusive Enantiomeric Form of the Soy Isoflavone Metabolite Produced by Human Intestinal Bacterial Flora. Am. J. Clin. Nutr. 2005, 81, 1072–1079. https://doi.org/10.1093/ajcn/81.5.1072.
- Jackson, R.L.; Greiwe, J.S.; Schwen, R.J. Emerging Evidence of the Health Benefits of S-Equol, an Estrogen Receptor β Agonist. Nutr. Rev. 2011, 69, 432–448. https://doi.org/10.1111/j.1753-4887.2011.00400.x.
- Wei, X.J.; Wu, J.; Ni, Y.D.; Lu, L.Z.; Zhao, R.Q. Antioxidant Effect of a Phytoestrogen Equol on Cultured Muscle Cells of Embryonic Broilers. Vitr. Cell Dev. Biol. Anim. 2011, 47, 735–741. https://doi.org/10.1007/s11626-011-9464-x.
- Choi, E.J.; Kim, G.H. The Antioxidant Activity of Daidzein Metabolites, O-Desmethylangolensin and Equol, in HepG2 Cells. Mol. Med. Rep. 2014, 9, 328–332. https://doi.org/10.3892/mmr.2013.1752.
- Messina, M. Soy and Health Update: Evaluation of the Clinical and Epidemiologic Literature. Nutrients 2016, 8, 754. https://doi.org/10.3390/nu8120754.
- Harland, J.I.; Haffner, T.A. Systematic Review, Meta-Analysis and Regression of Randomised Controlled Trials Reporting an Association between an Intake of circa 25 g Soya Protein per Day and Blood Cholesterol. Atherosclerosis 2008, 200, 13–27. https://doi.org/10.1016/j.atherosclerosis.2008.04.006.
- Wei, P.; Liu, M.; Chen, Y.; Chen, D.-C.; De-Cai, C. Systematic Review of Soy Isoflavone Supplements on Osteoporosis in Women. Asian Pac. J. Trop Med. 2012, 5, 243–248.
- Jing, Z.; Wei-Jie, Y. Effects of Soy Protein Containing Isoflavones in Patients with Chronic Kidney Disease: A Systematic Review and Meta-Analysis. Clin. Nutr. 2016, 35, 117–124. https://doi.org/10.1016/j.clnu.2015.03.012.
- Jayachandran, M.; Xu, B. An Insight into the Health Benefits of Fermented Soy Products. Food Chem. 2019, 271, 362–371. https://doi.org/10.1016/j.foodchem.2018.07.158.
- Franca Adriana, S.; Oliveira Leandro, S. Coffee and Its By-Products as Sources of Bioactive Compounds; Massey, J.L., Ed.; Nova Science Publishers, Inc.: New York, NY, USA, 2016; ISBN 978-1-63484-714-8.
- Clifford, M.N.; Jaganath, I.B.; Ludwig, I.A.; Crozier, A. Chlorogenic Acids and the Acyl-Quinic Acids: Discovery, Biosynthesis, Bioavailability and Bioactivity. Nat. Prod. Rep. 2017, 34, 1391–1421. https://doi.org/10.1039/c7np00030h.
- Perrone, D.; Farah, A.; Donangelo, C.M.; de Paulis, T.; Martin, P.R. Comprehensive Analysis of Major and Minor Chlorogenic Acids and Lactones in Economically Relevant Brazilian Coffee Cultivars. Food Chem. 2008, 106, 859–867. https://doi.org/10.1016/j.foodchem.2007.06.053.
- Langyan, S.; Yadava, P.; Sharma, S.; Gupta, N.C.; Bansal, R.; Yadav, R.; Kalia, S.; Kumar, A. Food and Nutraceutical Functions of Sesame Oil: An Underutilized Crop for Nutritional and Health Benefits. Food Chem. 2022, 389, 132990. https://doi.org/10.1016/j.foodchem.2022.132990.
- Pathak, N.; Rai, A.K.; Kumari, R.; Bhat, K. v. Value Addition in Sesame: A Perspective on Bioactive Components for Enhancing Utility and Profitability. Pharm. Rev. 2014, 8, 147–155. https://doi.org/10.4103/0973-7847.134249.
- Andargie, M.; Vinas, M.; Rathgeb, A.; Möller, E.; Karlovsky, P. Lignans of Sesame (Sesamum Indicum, L.): A Comprehensive Review. Molecules 2021, 26, 883. https://doi.org/10.3390/molecules26040883.
- Cha, J.W.; Piao, M.J.; Kim, K.C.; Yao, C.W.; Zheng, J.; Kim, S.M.; Hyun, C.L.; Ahn, Y.S.; Hyun, J.W. The Polyphenol Chlorogenic Acid Attenuates UVB-Mediated Oxidative Stress in Human HaCaT Keratinocytes. Biomol. Ther. 2014, 22, 136–142. https://doi.org/10.4062/biomolther.2014.006.
- Zang, L.-Y.; Cosma, G.; Gardner, H.; Castranova, V.; Vallyathan, V. Effect of Chlorogenic Acid on Hydroxyl Radical. Mol. Cell. Biochem. 2003, 247, 205–210. https://doi.org/10.1023/a:1024103428348.
- Kono, Y.; Kobayashi, K.; Tagawa, S.; Adachi, K.; Ueda, A.; Sawa, Y.; Shibata, H. Antioxidant Activity of Polyphenolics in Diets Rate Constants of Reactions of Chlorogenic Acid and Caffeic Acid with Reactive Species of Oxygen and Nitrogen. Biochim. Biophys. Acta 1997, 1335, 335–342. https://doi.org/10.1016/s0304-4165(96)00151-1.
- He, X.; Zheng, S.; Sheng, Y.; Miao, T.; Xu, J.; Xu, W.; Huang, K.; Zhao, C. Chlorogenic Acid Ameliorates Obesity by Preventing Energy Balance Shift in High-Fat Diet Induced Obese Mice. J. Sci. Food Agric. 2021, 101, 631–637. https://doi.org/10.1002/jsfa.10675.
- Ye, X.; Liu, Y.; Hu, J.; Gao, Y.; Ma, Y.; Wen, D. Chlorogenic Acid-Induced Gut Microbiota Improves Metabolic Endotoxemia. Front. Endocrinol. 2021, 12, 762691. https://doi.org/10.3389/fendo.2021.762691.
- Wang, Z.; Lam, K.L.; Hu, J.; Ge, S.; Zhou, A.; Zheng, B.; Zeng, S.; Lin, S. Chlorogenic Acid Alleviates Obesity and Modulates Gut Microbiota in High-Fat-Fed Mice. Food Sci. Nutr. 2019, 7, 579–588. https://doi.org/10.1002/fsn3.868.
- Huxley, R.; Man Ying Lee, C.; Barzi, F.; Timmermeister, L.; Czernichow, S.; Perkovic, V.; Grobbee, D.E.; Batty, D.; Woodward, M. Coffee, Decaffeinated Coffee, and Tea Consumption in Relation to Incident Type 2 Diabetes Mellitus. Arch. Intern. Med. 2009, 169, 2053–2063. https://doi.org/10.1001/archinternmed.2009.439.
- Mills, C.E.; Tzounis, X.; Oruna-Concha, M.J.; Mottram, D.S.; Gibson, G.R.; Spencer, J.P.E. In Vitro Colonic Metabolism of Coffee and Chlorogenic Acid Results in Selective Changes in Human Faecal Microbiota Growth. Br. J. Nutr. 2015, 113, 1220–1227. https://doi.org/10.1017/S0007114514003948.
- Sales, A.L.; dePaula, J.; Mellinger Silva, C.; Cruz, A.; Lemos Miguel, M.A.; Farah, A. Effects of Regular and Decaffeinated Roasted Coffee ( Coffea Arabica and Coffea Canephora ) Extracts and Bioactive Compounds on in Vitro Probiotic Bacterial Growth. Food Funct. 2020, 11, 1410–1424. https://doi.org/10.1039/C9FO02589H.
- Kamal-Eldin, A.; Moazzami, A.; Washi, S. Sesame Seed Lignans: Potent Physiological Modulators and Possible Ingredients in Functional Foods & Nutraceuticals. Recent Pat. Food Nutr. Agric. 2011, 3, 17–29. https://doi.org/10.2174/2212798411103010017.
- Dalibalta, S.; Majdalawieh, A.F.; Manjikian, H. Health Benefits of Sesamin on Cardiovascular Disease and Its Associated Risk Factors. Saudi Pharm. J. 2020, 28, 1276–1289. https://doi.org/10.1016/j.jsps.2020.08.018.
- Wu, W.-H.; Kang, Y.-P.; Wang, N.-H.; Jou, H.-J.; Wang, T.-A. Sesame Ingestion Affects Sex Hormones, Antioxidant Status, and Blood Lipids in Postmenopausal Women. J. Nutr. 2006, 136, 1270–1275. https://doi.org/10.1093/jn/136.5.1270.
- Oikawa, D.; Yamashita, S.; Takahashi, S.; Waki, T.; Kikuchi, K.; Abe, T.; Katayama, T.; Nakayama, T. (+)-Sesamin, a Sesame Lignan, Is a Potent Inhibitor of Gut Bacterial Tryptophan Indole-Lyase That Is a Key Enzyme in Chronic Kidney Disease Pathogenesis. Biochem. Biophys. Res. Commun. 2022, 590, 158–162. https://doi.org/10.1016/j.bbrc.2021.12.088.
- Zhu, X.; Zhang, X.; Sun, Y.; Su, D.; Sun, Y.; Hu, B.; Zeng, X. Purification and Fermentation in Vitro of Sesaminol Triglucoside from Sesame Cake by Human Intestinal Microbiota. J. Agric. Food Chem. 2013, 61, 1868–1877. https://doi.org/10.1021/jf304643k.
- Rothwell, J.A.; Perez-Jimenez, J.; Neveu, V.; Medina-Remón, A.; M’hiri, N.; García-Lobato, P.; Manach, C.; Knox, C.; Eisner, R.; Wishart, D.S.; et al. Phenol-Explorer 3.0: A Major Update of the Phenol-Explorer Database to Incorporate Data on the Effects of Food Processing on Polyphenol Content. Database 2013, 2013, bat070. https://doi.org/10.1093/database/bat070.
- Gitea, M.A.; Bungau, S.G.; Gitea, D.; Pasca, B.M.; Purza, A.L.; Radu, A.-F. Evaluation of the Phytochemistry–Therapeutic Activity Relationship for Grape Seeds Oil. Life 2023, 13, 178. https://doi.org/10.3390/life13010178.
- Walle, T. Bioavailability of Resveratrol. Ann. N. Y. Acad. Sci. 2011, 1215, 9–15. https://doi.org/10.1111/j.1749-6632.2010.05842.x.
- Francioso, A.; Mastromarino, P.; Masci, A.; d’Erme, M.; Mosca, L. Chemistry, Stability and Bioavailability of Resveratrol. Med. Chem. 2014, 10, 237–245. https://doi.org/10.2174/15734064113096660053.
- Wenzel, E.; Somoza, V. Metabolism and Bioavailability of Trans-Resveratrol. Mol. Nutr. Food Res. 2005, 49, 472–481. https://doi.org/10.1002/mnfr.200500010.
- Wu, B.; Basu, S.; Meng, S.; Wang, X.; Zhang, S.; Hu, M. Regioselective Sulfation and Glucuronidation of Phenolics: Insights into the Structural Basis of Conjugation. Curr. Drug Metab. 2011, 12, 900–916. https://doi.org/10.2174/138920011797470100.
- Song, X.; Liu, L.; Peng, S.; Liu, T.; Chen, Y.; Jia, R.; Zou, Y.; Li, L.; Zhao, X.; Liang, X.; et al. Resveratrol Regulates Intestinal Barrier Function in Cyclophosphamide-Induced Immunosuppressed Mice. J. Sci. Food Agric. 2022, 102, 1205–1215. https://doi.org/10.1002/jsfa.11458.
- Yao, M.; Fei, Y.; Zhang, S.; Qiu, B.; Zhu, L.; Li, F.; Berglund, B.; Xiao, H.; Li, L. Gut Microbiota Composition in Relation to the Metabolism of Oral Administrated Resveratrol. Nutrients 2022, 14, 1013. https://doi.org/10.3390/nu14051013.
- Wang, P.; Li, D.; Ke, W.; Liang, D.; Hu, X.; Chen, F. Resveratrol-Induced Gut Microbiota Reduces Obesity in High-Fat Diet-Fed Mice. Int. J. Obes. 2020, 44, 213–225. https://doi.org/10.1038/s41366-019-0332-1.
- Wang, P.; Wang, J.; Li, D.; Ke, W.; Chen, F.; Hu, X. Targeting the Gut Microbiota with Resveratrol: A Demonstration of Novel Evidence for the Management of Hepatic Steatosis. J. Nutr. Biochem. 2020, 81, 108363. https://doi.org/10.1016/j.jnutbio.2020.108363.
- Chen, M.; Hou, P.; Zhou, M.; Ren, Q.; Wang, X.; Huang, L.; Hui, S.; Yi, L.; Mi, M. Resveratrol Attenuates High-Fat Diet-Induced Non-Alcoholic Steatohepatitis by Maintaining Gut Barrier Integrity and Inhibiting Gut Inflammation through Regulation of the Endocannabinoid System. Clin. Nutr. 2020, 39, 1264–1275. https://doi.org/10.1016/j.clnu.2019.05.020.
- Zhang, B.; Xu, Y.; Lv, H.; Pang, W.; Wang, J.; Ma, H.; Wang, S. Intestinal Pharmacokinetics of Resveratrol and Regulatory Effects of Resveratrol Metabolites on Gut Barrier and Gut Microbiota. Food Chem. 2021, 357, 129532. https://doi.org/10.1016/j.foodchem.2021.129532.
- Korsholm, A.S.; Kjær, T.N.; Ornstrup, M.J.; Pedersen, S.B. Comprehensive Metabolomic Analysis in Blood, Urine, Fat, and Muscle in Men with Metabolic Syndrome: A Randomized, Placebo-Controlled Clinical Trial on the Effects of Resveratrol after Four Months’ Treatment. Int. J. Mol. Sci. 2017, 18, 554. https://doi.org/10.3390/ijms18030554.
- Timmers, S.; Konings, E.; Bilet, L.; Houtkooper, R.H.; van de Weijer, T.; Goossens, G.H.; Hoeks, J.; van der Krieken, S.; Ryu, D.; Kersten, S.; et al. Calorie Restriction-like Effects of 30 Days of Resveratrol Supplementation on Energy Metabolism and Metabolic Profile in Obese Humans. Cell Metab. 2011, 14, 612–622. https://doi.org/10.1016/j.cmet.2011.10.002.
- Walle, T.; Hsieh, F.; DeLegge, M.H.; Oatis, J.E.; Walle, U.K. High Absorption but Very Low Bioavailability of Oral Resveratrol in Humans. Drug Metab. Dispos. 2004, 32, 1377–1382. https://doi.org/10.1124/dmd.104.000885.
- Castaldo, L.; Narváez, A.; Izzo, L.; Graziani, G.; Gaspari, A.; di Minno, G.; Ritieni, A. Red Wine Consumption and Cardiovascular Health. Molecules 2019, 24, 3626. https://doi.org/10.3390/molecules24193626.
- Golan, R.; Shelef, I.; Shemesh, E.; Henkin, Y.; Schwarzfuchs, D.; Gepner, Y.; Harman-Boehm, I.; Witkow, S.; Friger, M.; Chassidim, Y.; et al. Effects of Initiating Moderate Wine Intake on Abdominal Adipose Tissue in Adults with Type 2 Diabetes: A 2-Year Randomized Controlled Trial. Public Health Nutr. 2017, 20, 549–555. https://doi.org/10.1017/S1368980016002597.
- Bresciani, L.; Favari, C.; Calani, L.; Francinelli, V.; Riva, A.; Petrangolini, G.; Allegrini, P.; Mena, P.; del Rio, D. The Effect of Formulation of Curcuminoids on Their Metabolism by Human Colonic Microbiota. Molecules 2020, 25, 940. https://doi.org/10.3390/molecules25040940.
- Chikara, S.; Nagaprashantha, L.D.; Singhal, J.; Horne, D.; Awasthi, S.; Singhal, S.S. Oxidative Stress and Dietary Phytochemicals: Role in Cancer Chemoprevention and Treatment. Cancer Lett. 2018, 413, 122–134. https://doi.org/10.1016/j.canlet.2017.11.002.
- Naeini, M.B.; Momtazi, A.A.; Jaafari, M.R.; Johnston, T.P.; Barreto, G.; Banach, M.; Sahebkar, A. Antitumor Effects of Curcumin: A Lipid Perspective. J. Cell Physiol. 2019, 234, 14743–14758. https://doi.org/10.1002/jcp.28262.
- Kunnumakkara, A.B.; Bordoloi, D.; Padmavathi, G.; Monisha, J.; Kishor Roy, N.; Prasad, S.; Aggarwal, B.B. Curcumin, the Golden Nutraceutical: Multitargeting for Multiple Chronic Diseases. Br. J. Pharmacol. 2017, 174, 1325–1348. https://doi.org/10.1111/bph.13621.
- Eke-Okoro, U.J.; Raffa, R.B.; Pergolizzi, J.V.; Breve, F.; Taylor, R. Curcumin in Turmeric: Basic and Clinical Evidence for a Potential Role in Analgesia. J. Clin. Pharm. 2018, 43, 460–466. https://doi.org/10.1111/jcpt.12703.
- Chen, C.Y.; Kao, C.L.; Liu, C.M. The Cancer Prevention, Anti-Inflammatory and Anti-Oxidation of Bioactive Phytochemicals Targeting the TLR4 Signaling Pathway. Int. J. Mol. Sci. 2018, 19, 2729. https://doi.org/10.3390/ijms19092729.
- Burge, K.; Gunasekaran, A.; Eckert, J.; Chaaban, H. Curcumin and Intestinal Inflammatory Diseases: Molecular Mechanisms of Protection. Int. J. Mol. Sci. 2019, 20, 1912. https://doi.org/10.3390/ijms20081912.
- Carlos-Reyes, Á.; López-González, J.S.; Meneses-Flores, M.; Gallardo-Rincón, D.; Ruíz-García, E.; Marchat, L.A.; Astudillo-De La Vega, H.; Hernández De La Cruz, O.N.; López-Camarillo, C. Dietary Compounds as Epigenetic Modulating Agents in Cancer. Front. Genet. 2019, 10, 79. https://doi.org/10.3389/fgene.2019.00079.
- Bahrami, A.; Amerizadeh, F.; ShahidSales, S.; Khazaei, M.; Ghayour-Mobarhan, M.; Sadeghnia, H.R.; Maftouh, M.; Hassanian, S.M.; Avan, A. Therapeutic Potential of Targeting Wnt/β-Catenin Pathway in Treatment of Colorectal Cancer: Rational and Progress. J. Cell. Biochem. 2017, 118, 1979–1983. https://doi.org/10.1002/jcb.25903.
- Adiwidjaja, J.; McLachlan, A.J.; Boddy, A.V. Curcumin as a Clinically-Promising Anti-Cancer Agent: Pharmacokinetics and Drug Interactions. Expert Opin. Drug Metab. Toxicol. 2017, 13, 953–972. https://doi.org/10.1080/17425255.2017.1360279.
- Hanai, H.; Iida, T.; Takeuchi, K.; Watanabe, F.; Maruyama, Y.; Andoh, A.; Tsujikawa, T.; Fujiyama, Y.; Mitsuyama, K.; Sata, M.; et al. Curcumin Maintenance Therapy for Ulcerative Colitis: Randomized, Multicenter, Double-Blind, Placebo-Controlled Trial. Clin. Gastroenterol. Hepatol. 2006, 4, 1502–1506. https://doi.org/10.1016/j.cgh.2006.08.008.
- Koosirirat, C.; Linpisarn, S.; Changsom, D.; Chawansuntati, K.; Wipasa, J. Investigation of the Anti-Inflammatory Effect of Curcuma Longa in Helicobacter Pylori-Infected Patients. Int. Immunopharmacol. 2010, 10, 815–818. https://doi.org/10.1016/j.intimp.2010.04.021.
- Di Mario, F.; Cavallaro, L.G.; Nouvenne, A.; Stefani, N.; Cavestro, G.M.; Iori, V.; Maino, M.; Comparato, G.; Fanigliulo, L.; Morana, E.; et al. A Curcumin-Based 1-Week Triple Therapy for Eradication of Helicobacter Pylori Infection: Something to Learn From Failure? Helicobacter 2007, 12, 238–243.
Disclaimer/Publisher’s Note: The statements, opinions and data contained in all publications are solely those of the individual author(s) and contributor(s) and not of MDPI and/or the editor(s). MDPI and/or the editor(s) disclaim responsibility for any injury to people or property resulting from any ideas, methods, instructions or products referred to in the content.
This entry is adapted from the peer-reviewed paper 10.3390/antiox12040880