1. Bulk Oil
Bulk oil is a heterogeneous system that comprises small levels of water. In addition, bulk oil contains various amphiphilic compounds that can entrap water molecules and produce reverse micelles in the bulk oil. These structures, known as association colloids, are primarily lamellar structures and reverse micelles. Various types of water or concentrations of surface-active compounds can result in various shapes of association colloids and, thus, impact lipid oxidation in different ways
[1]. Some surface-active compounds have low values of hydrophilic–lipophilic balance, namely monoacylglycerol (~3.4–3.8), diacylglycerol (~1.8), and free fatty acids (~1). These compounds are capable of creating reverse micelles in bulk oil. Phospholipids with a medium hydrophilic–lipophilic balance value (~8) can create reverse micelles and lamellar structures in bulk oil. Lipid hydroperoxides can reportedly accumulate at the interfacial region of reverse micelles
[2].
1.1. Role of Association Colloids on Bulk Oil Peroxidation
Association colloids can affect oxidation rate because they can produce oil–water interfaces where antioxidants and pro-oxidant compounds react with triacylglycerols
[3]. A list of possible pro-oxidants in lipid systems is presented in
Table 1. It is reported that phosphatidylcholine (PC) and phosphatidylethanolamine (PE) act as pro-oxidants in stripped peanut oil when their concentrations are beyond the critical micelle concentration (CMC). This pro-oxidant activity can be attributed to the presence of oil–water interfaces produced by phospholipid reverse micelles that can alter the location of amphiphilic and hydrophilic pro-oxidant compounds so that they approach the triacylglycerol substrate. In addition, phospholipids can decrease oil surface tension, which results in an enhanced oxygen diffusion rate in the oil
[4]. It has been shown that free fatty acids can affect the reverse micelle structure of DOPC (1,2-dioleoyl-sn-glycero-3-phosphocholine) in bulk oil. Oxidation experiments on lipid hydroperoxides and an evaluation of hexanal production showed that free fatty acids exhibit pro-oxidant effects in the absence or presence of DOPC. Free fatty acids can bind metal ions, render them more pro-oxidative, and accelerate the decomposition of lipid hydroperoxides. In addition, free fatty acids can decrease surface tension and enhance the diffusion rate of oxygen from the headspace into bulk oil
[5]. The association colloids formed by mixtures of surface-active compounds (dioleoylphosphatidylethanolamine, 1,2-dioleoyl-sn-glycero-3-phosphocholine, diolein, stigmasterol, and oleic acid) increase the lipid oxidation rate in a mixture of stripped corn oil/medium chain triglycerides
[6]. The fatty acid profile can affect the properties of association colloids and the oxidation rate of bulk oils. Homma et al.
[7] reported that the presence of association colloids in stripped high-oleic safflower oil and high-linoleic safflower oil did not have an effect on the lag phases of lipid hydroperoxides, nonanal, or hexanal formation. However, association colloids reduced the lag phases of both lipid hydroperoxides and propanal formation in fish triacylglycerols. Jo and Lee
[8] reported that propanal and hexanal enhanced the CMC of DOPC, while the CMC of DOPC decreased in the presence of nonanal. These observations indicate that aldehydes participate in producing association colloids in bulk oils. Furthermore, they stated that aldehydes could accelerate the rate of oxidation as they participate in the production of more association colloids.
Table 1. Pro-oxidant compounds in bulk oil and oil-in-water emulsion.
1.2. Effect of Association Colloids on the Efficiency of Antioxidants
Association colloids can impact the efficiency of antioxidant compounds in bulk oil
[15]. For instance, it has been reported that DOPC-reverse micelles improve the efficiency of Trolox (6-Hydroxy-2,5,7,8-tetramethylchroman-2-carboxylic acid) and α-tocopherol when applied at a low concentration (10 µM); however, their efficiency decreased at a high concentration (100 µM). In addition, hydrophobic α-tocopherol was less efficient than hydrophilic Trolox. This difference in the efficiency of α-tocopherol and Trolox can be attributed to the differences in their physical location on the DOPC-reverse micelles
[1]. In addition, when α-tocopherol and canolol were used at concentrations of 100 µM in rapeseed oil, their antioxidant efficiency decreased in the presence of DOPC association colloids during the storage period. This decrease in antioxidant efficiency could be related to the formation of amphiphilic compounds during autoxidation, which can impact the structure of reverse micelles and modify antioxidant efficiency
[16]. According to Laguerre et al.
[15], the efficiency of hexadecyl chlorogenate decreased when DOPC was incorporated into corn oil at a concentration higher than its CMC (200 µM). In contrast, DOPC did not alter the efficiency of chlorogenic acid. On the contrary, the antioxidant efficiency of chlorogenic acid decreased when a combination of DOPC (200 µM) and water (∼400 ppm) was incorporated into the corn oil. However, a combination of DOPC and water did not alter the efficiency of hexadecyl chlorogenate. Adding water to stripped corn oil containing DOPC produced reverse micelles with a water core. This water core can enhance the affinity of free chlorogenic acid for locating into the reverse micelles, where it can interact with water-soluble metal ions or metal ions attached to anionic phospholipids, thereby enhancing the pro-oxidant effect of the transition metals
[15].
2. O/W Emulsion
O/W emulsions usually oxidize faster than bulk oils. The faster oxidation rate of O/W emulsions is related to several reasons. First, the presence of the interfacial region between the water and oil phase is assumed to promote contact between pro-oxidant compounds present in the water phase and unsaturated lipids. In addition, a high interfacial region could enhance the accessibility of the oil phase to the oxygen in the water phase. Second, the process of emulsion production can enhance oxidation by incorporating oxygen, the direct formation of free radicals via acoustic cavitation in the case of sonication or by overheating due to shear stress
[17][18][19]. The physicochemical properties of the interfacial region, transfer of oxidants and antioxidants between oil droplets, lipid and aqueous phase components, the size of oil droplets, pH, oxygen concentration, and chemical structures of lipids can all contribute to the impact on the oxidation of O/W emulsions (
Figure 1)
[20][21].
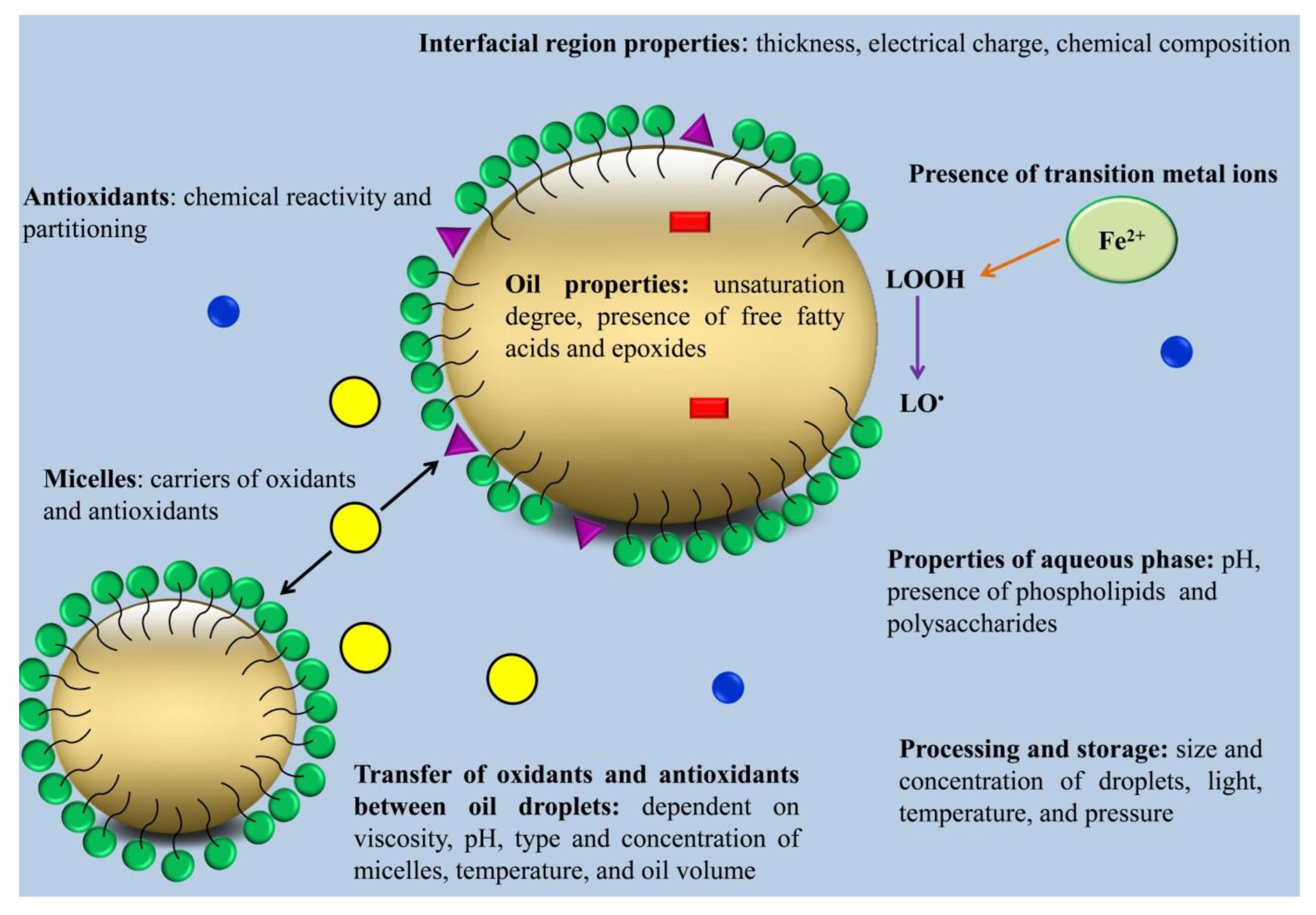
Figure 1. Factors affecting O/W emulsion oxidation (hydrophobic antioxidants: red rectangle; hydrophilic antioxidants: blue circle; amphiphilic antioxidants: purple triangle; and micelles: yellow circle ) (adopted from Ref.
[22]).
2.1. Physicochemical Properties of the Interfacial Region
Physicochemical properties of the interfacial region can impact emulsion oxidation because they affect lipid hydroperoxides and their capability to interact with metal ions
[23].
The thickness of the interfacial region can strongly affect the oxidation rate of emulsions because the lipid oxidation rate is influenced by the interactions of unsaturated lipids in the oil droplets with pro-oxidants present in the water phase. Therefore, inhibiting the transfer of pro-oxidants in the water phase toward the oil droplets can reduce lipid oxidation. This can be achieved by forming thick coatings around the oil droplets
[17][24][25].
The charge of the interfacial region is another factor that can affect the oxidation rate of O/W emulsions. The charge of the interfacial region depends on surfactant type, other oppositely charged components in the emulsion that can attach to the emulsion droplets surface, ionic strength, and pH
[26][27][28]. The negative charge of the interfacial region can enhance the oxidation rate by attracting metal ions towards the oil phase
[29]. The pH of the emulsion can alter the charge of the proteins. The charge of the interfacial region stabilized by proteins is negative at pH values higher than the isoelectric point of proteins. Therefore, proteins can enhance the oxidation rate of emulsions at high pH values
[30].
2.2. Oil and Aqueous Phase Components
Free fatty acids that remain in the oil phase after being refined can affect the lipid oxidation rate. In this regard, Waraho et al.
[11] stated that oleic acid reduced oxidative stability via enhancing the negative charge of emulsion droplets. The pro-oxidant effects of unsaturated fatty acids were in the order of linolenic < linoleic < oleic. This pro-oxidant effect is related to the ability of free fatty acids to attract pro-oxidant metal ions and to co-oxidize triacylglycerols in bulk oil
[31].
Phospholipids can both inhibit and promote lipid oxidation in the oil phase. The pro-oxidant effects of phospholipids are due to their anionic physical structures that can attract pro-oxidant metal ions. Meanwhile, their inhibitory effect against lipid oxidation is due to their ability to increase the affinity of antioxidants to locate at the interfacial region, decompose hydroperoxides, and chelate metal ions
[12].
Proteins in the water phase can decrease the oxidation rate in O/W emulsions using several different mechanisms. For example, cysteine, tryptophan, and tyrosine amino acids can exhibit radical scavenging and metal-chelating activities at pH values higher than the isoelectric point of the protein
[23].
Some polysaccharides, such as glycoproteins, gum Arabic, and soluble soybean polysaccharides, can enhance O/W emulsion oxidative stability through interacting with metal ions and free radicals. In addition, polysaccharides can lower the diffusion rate of pro-oxidant metal ions and oxygen toward the interfacial region by enhancing the viscosity of the water phase
[32].
2.3. Size of Oil Droplets
The size of oil droplets can alter lipid oxidation rate by affecting the contact area between unsaturated fatty acids and pro-oxidants in the water phase. It is expected that small droplets can oxidize faster because they can create a larger contact area between unsaturated fatty acids and pro-oxidants in the water phase
[33]. However, some researchers have indicated that O/W emulsions containing small droplets have higher oxidative stability than those containing larger droplets
[34]. These contradictory results show that adjusting the size of oil droplets can alter other parameters affecting the rate of lipid oxidation. For example, droplets of different sizes have different curvatures in the droplet surface, which can change the packing properties of the emulsifiers. In addition, when lipid concentration is constant, reducing the size of droplets can enhance the concentration of the interfacial emulsifier and decrease the distance between oil droplets. This reduction in the distance between oil droplets is expected to enhance the exchange of lipid oxidation products, transition metal ions, and antioxidants between oil droplets.
[22]. Increasing the transfer rate of antioxidants between oil droplets can enhance their efficiency in O/W emulsion and reduce oxidation rate, while increasing the transfer rate of lipid oxidation products and transition metal ions can enhance the oxidation rate
[35]. Taken together, reducing oil droplet size in different lipid systems does not always have the same effect on lipid oxidation. Depending on the type of lipid system and the molecular species present in the system, the reduction in oil droplets may decrease or increase the oxidation rate.
2.4. Transfer of Oxidants and Antioxidants between Oil Droplets
Besides the importance of the physicochemical properties of the interfacial region, the transport of lipid oxidation products, transition metal ions, and antioxidants is a crucial event that is often ignored in the determination of the oxidation rate in emulsions. Three mechanisms of mass transfer in O/W emulsions include (I) diffusion, (II) collision–exchange–separation, and (III) micelle-assisted transfer. According to the diffusion mechanism, molecules diffuse from one oil droplet to another through the water phase. According to the collision–exchange–separation mechanism, when oil droplets collide with each other, they transfer from one particle to another. According to the micelle-assisted transfer mechanism, molecules solubilize in micelles within the water phase and then transfer between lipid droplets
[20]. Three types of compounds can be produced during lipid oxidation based on their diffusion properties as follows: (I) water-soluble compounds, such as carbonyl compounds; (II) surface-active compounds (e.g., LOOHs); and (III) hydrophobic compounds (e.g., lipoperoxy radicals)
[36]. Water-soluble compounds can transfer between oil droplets through the diffusion mechanism, while hydrophobic compounds can be transferred only through the collision–exchange–separation mechanism. Transfer of hydrophobic compounds through the collision–exchange–separation mechanism is slower than that of water-soluble compounds through the diffusion mechanism. When micelles are present in the water phase, the micelle-assisted transfer mechanism occurs that can result in a drastic increase in the transfer rate of hydrophobic compounds between oil droplets. The rate of collision–exchange–separation and micelle-assisted transfer mechanisms depends on the size and concentration of lipid droplets and micelles. In O/W emulsions that contain a high concentration of micelles and large droplets, the micelle-assisted transfer mechanism would be faster than the collision–exchange–separation mechanism
[20]. The ability of molecules produced during lipid oxidation to transfer from one oil droplet to the other one depends on their stability in the system. For instance, peroxyl radicals induced by AMVN (2,2′-azobis (2,4-dimethylvaleronitrile) could transfer between oil droplets
[37], while no transfer was observed for alkoxyl radicals produced by di-tertbutyl peroxide
[38]. This could be related to the shorter life span of alkoxy radicals (10
−6 s) than peroxyl radicals (0.5–7 s), which limits their transfer from one oil droplet to another one
[37][38]. A peroxyl radical can cross much longer distances (0.14 mm in a non-viscous medium and 0.2 × 10
−3 mm in a viscous one) than alkoxyl radicals (10
−4 mm in a non-viscous medium and 10
−7 mm in a viscous one)
[20]. LOOH molecules have a higher half-life than their radical homologs (LOO•) and are able to transfer between oil droplets. During lipid oxidation, the formation of lipid hydroperoxides micelles in the water phase of O/W emulsions can shift the transfer mechanism of lipid oxidation products from collision–exchange–separation to micelle-assisted transfer, which results in a sudden transition from the initiation stage to the propagation stage. The initiation stage is a transfer-controlled rate, meaning that that the rate of lipid oxidation is limited only by how fast oxidants can transfer from the oxidized oil droplet to the other one. Meanwhile, the propagation stage is a reaction-limited rate, meaning that the time required for oxidants to encounter an oxidizable substrate suddenly becomes negligible compared to the time required for the reaction to occur. In the presence of excess amounts of surfactants in the system, competitive adsorption processes in mixed micelles between surfactants and LOOH molecules may occur.
2.5. Surfactant Micelles
When a surfactant is used at concentrations higher than its CMC value, surfactant micelles are formed in the water phase of emulsions. These micelles can rapidly exchange lipid hydroperoxides, antioxidants, and metal ions by interacting with other micelles, with the water phase, or with oil droplets. In addition, surfactant micelles can form co-micelles with other surface-active agents and change the partitioning of emulsion components among the interfacial region, oil droplets, and aqueous phase
[39]. Surfactant micelles can contribute to the mass transport of oxidation products among the oil droplets; therefore, they can cause an increase or decrease in oxidation rate. In this regard, Raudsepp et al.
[37] indicated that radicals that are produced inside oil droplets could contribute to the initiation of lipid oxidation in neighboring oil droplets when surfactant micelles exist in the solution. In some cases, surfactant micelles can reduce O/W emulsion oxidation
[40]. It has been reported that surfactant micelles can partition iron out of the oil droplets, thereby reducing the oxidation rate
[41]. Additionally, when surfactant micelles are present, lipid hydroperoxides can partition out of the oil droplets, thereby reducing the oxidation rate
[39]. Furthermore, surfactant micelles can increase the efficiency of highly hydrophobic antioxidants by favoring their partitioning at the interfacial region
[42].
2.6. pH
The pH of the water phase can affect the charge of the oil droplets, the solubility of metal ions, and the chemical reactivity of antioxidants, metal ions, and chelators
[9][43][44]. The lipid oxidation rate in emulsions containing tannic acid increased in response to a decrease in pH from 3 to 7, which can be attributed to the decrease in the antioxidant capacity of tannic acid where acidic pH exists. In addition, acidic pH can increase the solubility and activity of the metal ions in the water phase
[45]. Furthermore, acidic pH can reduce the metal-chelating capacity of the tannic acid
[43]. It has been suggested that anionic polysaccharides, pectin, and xanthan gum have higher antioxidant activity at pH 3.5 than at pH 7. Xanthan gum was a better antioxidant than pectin at pH 3.5, while it showed a pro-oxidant effect at pH 7. When pH is acidic, anionic polysaccharides can bind to cationic metal ions, thereby reducing the pro-oxidant effect of metal ions
[46].
This entry is adapted from the peer-reviewed paper 10.3390/foods12061191