1. Structural Features and Physical Properties of TiO2 Nanoparticles
Understanding the fundamental properties of semiconductor materials governing their photoelectric performance (such as their crystal structure, lattice parameters, and optical and electronic properties) is essential to optimize their performance for photocatalytic applications. This section introduces the fundamentals underlying the photocatalytic performance of nanostructured TiO2.
1.1. Crystal Structures of TiO2
Morphology and the crystal structure of TiO
2 principally determine its photocatalytic activity. Therefore, essential factors for TiO
2 photocatalytic activity are crystallite size and the specific surface area
[1][2][3]. TiO
2 nanostructures with different shapes and titania-based nanocomposites have attracted much attention in research due to their diverse physicochemical characteristics. The 1D TiO
2 nanostructures have gained more attention compared to their 0D and 2D counterparts due to the higher aspect ratio, increased surface area, and efficient electronic charge properties
[4]. TiO
2 exists in two tetragonal forms (rutile and anatase) and one rhombic form (brookite),
Figure 1 [5][6][7][8]. Brookite is difficult to obtain in laboratory conditions, while rutile and anatase are easily prepared. As a bulk material, rutile is the stable phase; however, solution-phase preparation methods for TiO
2 generally favor the anatase structure. These observations are attributed to two main effects: surface energy and precursor chemistry. It has been found that the surface energy of anatase is lower than those of rutile and brookite
[8]. The concept of surface energy accurately explains the observed crossover size of about 30 nm where anatase nanoparticles transform to rutile
[9]. Secondly, the crystal structure stability has been explained based on a molecular picture. The precursor chemistry determines the nucleation and growth of the different polymorphs of TiO
2, which depends on the reactants used
[10][11]. A complicating factor in understanding nanoparticle formation is the multitude of experimental conditions used to synthesize the different TiO
2 phases, making it challenging to compare mechanisms
[8][12].
Figure 1. 3D visualization crystal structures of TiO
2 using visualization for electronic and structural analysis (VESTA)
[13].
These polymorphs, anatase, and rutile, exhibit different properties and, consequently, different photocatalytic performances. Of the two tetragonal forms mentioned, the anatase form shows significantly higher photocatalytic activity than rutile due to the higher presence and nature of surface hydroxyl groups. Transformation of anatase form to rutile happens at elevated temperatures of 700–1000 °C. Anatase form is stable at lower temperatures (it occurs in the form of a pyramidal crystal structure). At the same time, rutile (needle-shaped) is dominant during the synthesis at high temperatures
[14][15][16]. Anatase has a higher energy gap, which additionally contributes to the photocatalytic activity of this form. The energy gap of anatase is 3.20 eV, while the rutile energy gap is 3.02 eV
[17][18].
TiO
2 Degussa P25 is the most frequently used commercial product containing 75% of anatase form and 25% of rutile form
[19][20]. The mentioned mixture exhibits outstanding photocatalytic performance and superiority compared with other TiO
2 [21]. The predominant form of titania used is anatase. It was found that anatase is the most photocatalytic active form within TiO
2 polymorphs. TiO
2 Hombikat is a pure anatase form of photocatalyst
[19]. TiO
2 also appears in another form named TiO
2 Wackherr “oxide de titan standard”, which contains 100% of anatase form, exhibits interesting characteristics related to photocatalytic application, and is more efficient than TiO
2 Degussa P25
[22][23][24]. The main characteristic of this photocatalyst, when compared with TiO
2 Degussa P25, is a lower scattering of radiation in the UV area, which is most likely the consequence of the greater size of particles. Particle size is a crucial factor affecting the performance of nano-photocatalytic materials. The greater size of TiO
2 Wackherr particles than TiO
2 Degussa P25 results in a lower specific surface area. Particles with a lower specific surface area usually exhibit low photocatalytic activity. However, they can be more efficient in photocatalysis, where optical properties and low scattering are significant
[24][25].
1.2. Electronic Structure of TiO2
Understanding the electronic properties of semiconductor materials, including their band structure, nonequilibrium carrier concentration, carrier mobility, and lifetime is essential to achieve inflection of charge carrier behavior and enhancing photocatalytic performance. Against this background, electronic properties of anatase, rutile, and brookite TiO
2 phases were introduced
[26]. The current understanding of the electronic structures of TiO
2 is mainly based on the results of independent and combined theoretical calculations, usually in the framework of the density functional theory (DFT)
[6] and experimental techniques (e.g., synchrotron radiation photoelectron spectroscopy, UV-vis spectroscopy, ultraviolet photoelectron spectroscopy, and photoluminescence)
[27][28].
The application of various computational methods in elucidating the photocatalytic properties of novel TiO
2-based materials is essential. Namely, applying the DFT approach enables researchers to predict the band gap of newly designed materials and focus their attention on synthesizing materials with target photocatalytic properties. The computational aspect of band-gap engineering is critical in developing new photocatalytic materials, as this parameter is essential for practical applications
[29]. Additionally, the DFT approach, in combination with carefully selected density functionals and basis sets, is an excellent tool for understanding the light absorption properties of molecules that are degraded by photocatalysts, helping scientists to easier identify the degradation mechanism
[30].
Regarding the computational design of novel materials, the DFT approach offers the best cost–efficiency ratio
[31]. This flexible theoretical approach allows researchers to elucidate the structural and electronic properties of photocatalytic materials by analyzing how various structural alterations influence the electronic subsystem of nanomaterials.
However, the DFT approach’s severe drawback is that it severely underestimates the band gap values due to self-interaction error
[32][33][34][35]. Considering the fundamental importance of band gaps in photocatalysis, this is a significant challenge in designing novel compounds to be applied in this area. In general, this issue can be tackled in two main ways. One approach is to create specially designed density functionals that reproduce electron density adequately. The second approach is to use the existing density functionals and implement specific corrections within them. Both directions have positive and negative sides. For example, specially designed hybrid density functionals yield outstanding results in accuracy, but they are computationally much more demanding and are usually applicable only for single-point band structure calculations. Conversely, corrected density functionals offer improved results over conventional density functionals, with computational costs slightly more demanding than traditional density functionals.
Considering the application of hybrid functionals for band structure calculations, it is worth mentioning the importance of the HSE06 hybrid functional
[36]. Recent studies have confirmed this function’s importance in predicting band gaps of different materials with small, intermediate, and large values of this critical parameter
[37][38]. However, in terms of computational costs, hybrid density functionals may still be unavailable to significant number of research groups, which may apply the computationally affordable DFT + U method
[39]. This method adds a Hubbard-like term to the Hamiltonian to account for on-site interactions. The simplest version of the DFT + U approach relies on only one parameter—on-site Coulomb term (
U). However, this method can be improved by incorporating the site exchange term
J.
U and
J parameters can be obtained through ab initio applications. Still, they are frequently obtained empirically by testing a range of values. So far, many research studies have reported the values of these parameters for certain types of materials, so a literature survey is warmly recommended to find good starting values.
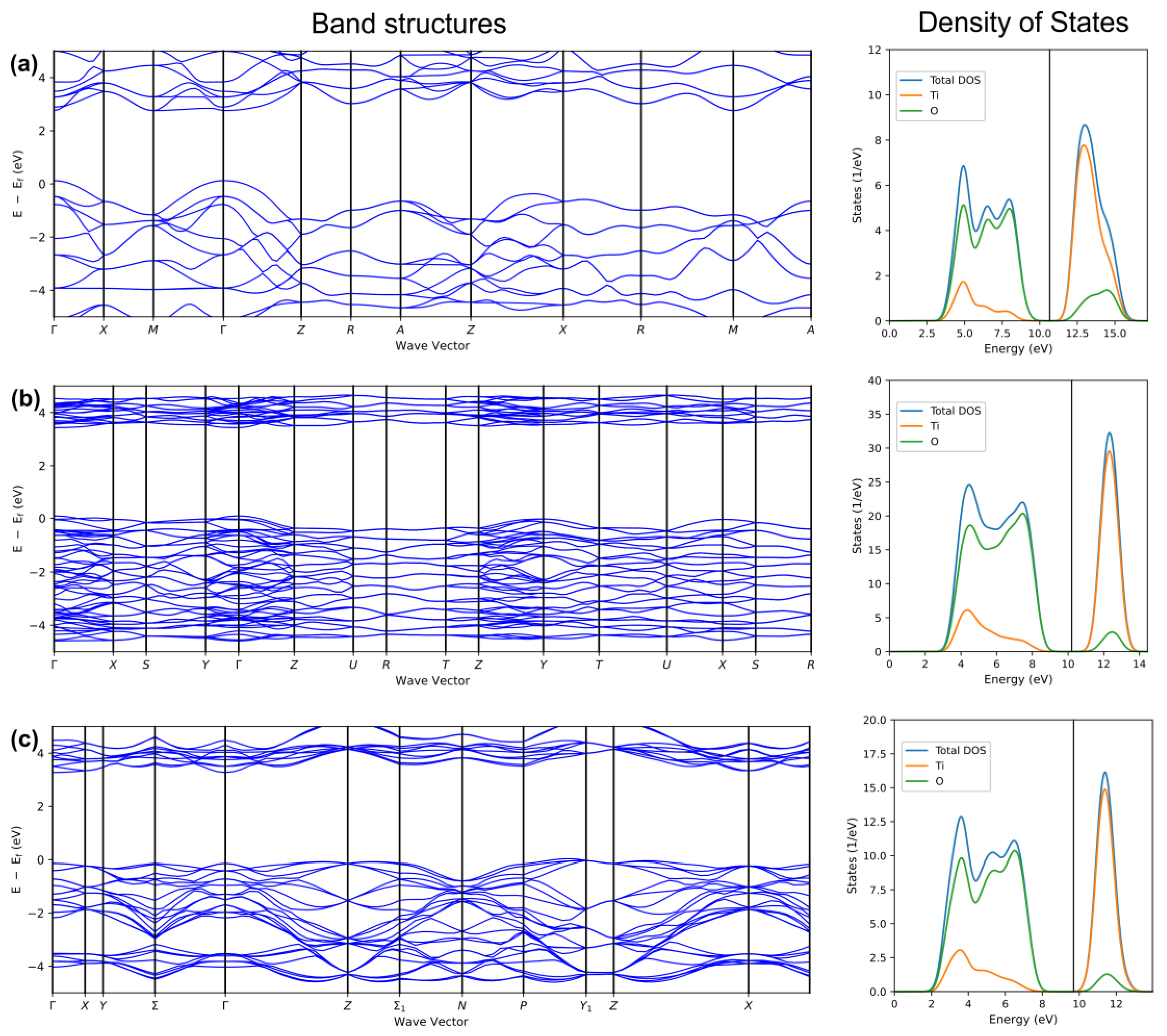
Figure 2. Band structures (left panel) and projected density of states (right panel) for the (a) rutile), (b) brookite, and (c) anatase polymorphs of TiO2, as obtained by DFT+U calculations (PBE functional, GBRV pseudopotentials, , ) in Quantum Espresso program as implemented in Schrödinger Materials Science Suite 2022-4.
In
Figure 2, the band structures and density of states as obtained by applying the DFT + U method are presented, with
U taking the value of 7.8 eV and
J taking the value of 1 eV, as reported in studies by
[40][41]. Inspection of band structures shows that the application of the DFT + U method provides results for band gaps that are in agreement with experimental results, which will be mentioned somewhat later. The density of states figures also show that the conduction band consists of Ti states, while valence band (VB) consists of O states. As reported in reference by Li et al.
[42], CB consists of Ti
3d states, while VB consists of O
2p states.
Ordinarily, TiO
2 phases, especially anatase, show good insulation in their ideal stoichiometric states due to their wide bandgap
[43]. However, certain types of point defects are unavoidably introduced during the preparation process. These defects might be interstitial titanium ions (T
3+), oxygen point defects, and substituted ions and can notably affect the charge carrier behavior, band structure, and, eventually, photocatalytic performance
[44]. The characteristics of defects (e.g., concentration, type, distribution, and dimension) and their influence on the photoinduced charge carriers in TiO
2 can be various
[45]. Point defects can induce the generation of defect states, the position generally influenced by the surface and phase. For instance, the defect states of rutile-TiO
2 (110) and anatase-TiO
2 (101) are found at ≈0.8–1.0 eV and ≈0.4–1.1 eV, of which both are below the CB edge
[46]. In the brookite phase, both rutile-like (Y-shaped) and anatase-like (T-shaped) OTi
3 building blocks exist, inducing the O
2p in VB to present characteristics from both tetragonal phases
[6]. Considering the application of hybrid and recently developed density functionals, the study by Dharmale et al. is worth mentioning. In their work, electronic properties such as effective mass, the partial density of states, the total density of states, and the band structure of brookite TiO
2 have been studied by applying using seven exchange-correlation functionals, including the already-mentioned HSE06
[47]. Band structures and projected density of states for the rutile), brookite, and anatase polymorphs of TiO
2 are shown in
Figure 2.
1.3. Optical Properties of TiO2
A semiconductor’s optical properties (e.g., photoconductivity, dielectric constant, refractive index, extinction coefficient, reflectivity, absorption coefficient, and loss function) are related to its bandgap
[48]. TiO
2 is commonly known as a wide-bandgap semiconductor with high susceptibility to UV light
[49][50]. The optical absorption in the visible and near-infrared regions is insignificant because photons in the visible region do not have intrinsic excitation of carriers. When the electrons (e
−) in the VB are exposed to UV radiation, they are excited to the CB, leaving holes (h
+) in the VB
[51][52][53]. The CB e
− is now in a purely 3D state, and the possibility of transition of e
− to the VB is reduced due to the difference in parity. Therefore, the probability of e
−–h
+ recombination is reduced
[54]. Consequently, separating energy between these two states defines the sensitivity of TiO
2 towards the light in the UV range. However, the optical properties at the surface differ considerably from those of the bulk material, providing extensive opportunities to optimize further the photoelectric and optical properties of TiO
2 [55].
The bandgap energies (i.e., optical absorption edges) of rutile, anatase, and brookite TiO
2 are estimated to be ≈3.00, 3.21, and 3.13 eV at room temperature, respectively. The photon energy values of optical absorption edges of rutile and anatase increase with the crystal growth temperature decrease
[8]. Overall, rutile and anatase bandgaps in bulk are considered to be indirect
[56]. Detailed examinations of rutile TiO
2 at temperature 1.6 K discover an anisotropic optical response characterized by a direct forbidden transition at ≈3.06 eV. At the same time, the bandgap near the edge is prevailed by an indirect transition. The direct bandgap transition of anatase occurs at ≈3.8–4.0 eV
[57]. Additionally, the orthorhombic TiO
2 brookite bandgap energy has been experimentally determined to be 3.1–3.4 eV. This is a biaxial material with three independent components other than the dielectric tensor of the uniaxial rutile and anatase materials
[8][58]. The significant value of the energy gap limits the exceptional photocatalytic characteristics of TiO
2. Namely, the photon energy should be high enough to excite the TiO
2 particles.
In recent experimental and theoretical investigations, efforts have been made to improve the optical properties of TiO
2 by increasing its photosensitivity and identifying the correlation between its surface, interfacial, and microstructural characteristics and the corresponding mechanisms crucial to its photoelectric properties
[59][60]. Generally used strategies to enhance the optical properties of TiO
2 include element doping
[61][62] coupled with other semiconductors to form heterojunctions
[63][64], synthesis of nanostructures with particular morphologies
[65], surface sensitization to improve optical characteristics using organic dyes, metal nanostructures or metal complexes
[66] and so on.
The doping technique can be explained as the deliberate addition of impurities into a semiconductor material (
Figure 3). To enhance TiO
2 catalytic activity under visible light, metal/non-metal-doped TiO
2 structures have been extensively studied
[67]. Adding metal/non-metal increases oxygen vacancies and reduces the band gap energy, resulting in higher photocatalytic activity
[68]. This could be useful in wastewater treatment for the photocatalytic degradation of organic compounds under visible and UV light radiation. Many different metals, such as Pd
[69], Pt
[70], Au, Ag
[71], Ce
[72], Sr
[73], V
[74] and so on, have been employed for the preparation of metal-doped TiO
2 catalysts.
Figure 3. Illustrating the generation of photoinduced e− and h+ in (a) pure TiO2, (b) metal-doped TiO2, (c) non-metal-doped TiO2, and (d) coupling TiO2 with the metal oxides.
The sol–gel method is often used in the synthesis, where the photocatalyst is doped with metals such as Fe, Ni, Cr, V, La, Nd, and Sm. The sol–gel process represents one of the versatile methods for preparing nano-dimensional materials. Incorporating an active dopant allows the doped element to interact directly with the support, which is why the material has catalytic or photocatalytic properties. When metal nanoparticles are doped into the TiO2, a new energy level or an interband state is produced in the band gap close to the CB arising from the partially filled d orbitals of the doping metal ions modifying the electronic structure and hence lowering the band gap (Figure 3b).
Although metal-ion doping decreases the energy gap of TiO
2, the aforementioned metal ions can also act as recombination centers for electrons and holes, thus reducing the overall activity of the TiO
2 [75]. In recent years, non-metals such as C, N, P, S, and B have been the best candidates for obtaining the desired band-gap narrowing of TiO
2 [76]. It was shown that non-metal doped TiO
2 shows significant catalytic activity under visible light radiation
[77]. Several researchers have depicted that TiO
2 doping with non-metal ions improve photocatalytic efficiency compared to metals. Doping with cations changes the morphology of the photocatalysts and the photocatalytic activity. It also affects the photocatalyst’s electronic structure. Non-metal dopants influence the VB of TiO
2 through interactions with 2p e
− of O and form an impurity level above the VB. Distinct non-metals, including N, C, S, F, B and so on, are used as dopants and have shown promising outcomes in recent research studies. Nitrogen doping in TiO
2 has gained considerable attention due to its ability to narrow the band gap and promote the electron–hole pair transfer mechanism (
Figure 3c). It has been observed that in the case of doping with lighter elements such as N, C, S, or B at substitutional sites of TiO
2, lower atomic number elements with smaller effective nuclear charge appear at a higher localized energy level in the band gap. Doping TiO
2 by carbon stabilizes anatase TiO
2, enhances its conductivity, and extends the pollutant adsorption on the surface of TiO
2 [78].
Žener et al.
[68] showed that doping, co-doping, and modifying TiO
2 samples with nitrogen, sulfur, and platinum increased their photocatalytic activity by up to 6 times. XRD measurements revealed that the replacement of HCl with H
2SO
4 during sol–gel synthesis reduced the size of the crystallites from ~30 nm to ~20 nm, increasing the surface area. This is consistent with the samples’ photocatalytic activity and the photocatalysts’ measured photocurrent behavior
[68].
Coupling TiO
2 with the metal oxides increases the charge carrier separation and thereby increases the lifetime of the charge carriers
[79]. When the coupled catalyst, consisting of TiO
2 and a semiconductor, is irradiated, both the TiO
2 and the semiconductor will excite electrons from VB to the CB using UV and Visible irradiation. TiO
2 and the semiconductor configuration for coupling are crucial for the enhancement activity. The CB of TiO
2 should be more favorable than the corresponding band of the semiconductor, and the VB should be more cathodic than that of TiO
2. The e
− from the CB of the semiconductor migrates to the CB of TiO
2 and increases the concentration of electrons at the TiO
2 conduction band. At the same time, the h
+ generated at the VB of TiO
2 will be transferred to the VB of the semiconductor, increasing the concentration of h
+ in the coupled semiconductor/TiO
2 (
Figure 3d)
[80][81][82]. Couplings of TiO
2 with a metal oxide such as ZnO
[83], SiO
2 [84], Cu
2O
[85], Bi
2O
3 or ZnMn
2O
4 [86], graphene
[87] and so on. are reported for many photocatalytic applications including organic pollutant degradation, water splitting, pharmaceutical degradation and so on. TiO
2–ZnO binary oxide systems containing various molar ratios of TiO
2–ZnO were prepared using a sol–gel method. It was reported that the crystalline structure, thermal stability, and porous structure parameters were determined by the molar ratio of TiO
2 to ZnO and the calcination process for the most part. TiO
2–ZnO showed high photocatalytic activity towards the degradation of C.I. Basic Blue 9, C.I. Basic Red 1, and C.I. Basic Violet 10 dyes
[83]. TiO
2 nanoparticles synthesised via the acid-catalysed sol–gel method were used to prepare coupled TiO
2/SiO
2 mesoporous materials were prepared by deposition of TiO
2 nanoparticles. TiO
2/SiO
2 showed photocatalytic activity towards the photodegradation of rhodamine 6G in aqueous solution using UV radiation
[84]. One of the noticed studies focuses on TiO
2 nanocomposite with graphene as photocatalyst, one of the most prominent representatives of carbon nanostructures. Coupling TiO
2 with graphene proved to be beneficial, as the higher efficiency in photocatalysis has been observed compared to that of TiO
2 alone. Graphene sheets are thought to act as an electron acceptor that enables the separation and transfer of photogenerated electrons during TiO
2 excitation, simultaneously reducing recombination of electron–hole pairs
[87]. Wang et al. reported one of the first studies where TiO
2 (P25)-graphene nanocomposites were used for photocatalytic degradation of methylene blue. Further research has led to the photodegradation of many organic pollutants by these materials
[88].
2. Practical Application
The possibility to activate catalysts with sunlight and recent advances in synthesis methods of the catalyst with desirable band gaps opened the opportunity to design prominent solar collectors where photochemical processes are promoted with the absorption of sunlight. While for solar thermal processes, it is essential to collect as many as possible photons of all wavelengths, for the solar photochemical process, it is crucial to collect only high-energy short-wavelength photons, which are responsible for the initiation of photochemical processes. Usually, for the initiation of solar photochemical processes, UV or near UV sunlight is necessary. However, there are some cases where the sunlight of up to 580 nm can be employed, while 600 nm and higher wavelengths are not usable for these purposes
[89]. Initially, photoreactors for photochemical applications were based on line-focus parabolic-trough concentrators. This hardware already existed for solar thermal applications and could be easily modified for photochemical processes. The first European facility for water detoxification based on solar photochemical processes was established in Spain by The Centre for Energy, Environmental and Technological Research. Twelve PTCs were used herein while non-concentrating collectors became popular since the influence of concentration and solar tracking does not reduce their efficiency.
Researchers from the Institute of Science and Technology for Ceramics, Italy, developed a TiO
2-coated fabric to be used as a photocatalyst agent to degrade Rhodamine B (RhB) in water. They implemented the obtained photocatalytic materials in a 6 L capacity semi-pilot plant. They evaluated the degradation of RhB dye, simulating the water pollution. The good results encouraged the scale-up of the 6 L semi-pilot plant up to the 100 L pilot plant built
[90].
Biologically pretreated industrial wastewater from the factories of the Volkswagen AG in Wolfsburg (Germany) and Taubate (Brazil) has been treated in laboratory conditions with great success, after which a pilot plant was installed in the Wolfsburg factory in 1998
[91]. Another project, called “SOLARDETOX”, is worth mentioning. The name is an abbreviation for Solar Detoxification Technology for the Treatment of Industrial Non-Biodegradable Persistent Chlorinated Water Contaminants. This project aimed to design and develop a commercial non-concentrating solar detoxification system using neither collecting nor non-collecting collectors but compound parabolic collector technology (CPC), having a concentration ratio equal to 1. CPCs present a particular class of solar collectors produced in the shape of two meeting parabolas. The CPC collector belongs to a non-imaging class of collectors and is considered one of the types with the highest possible concentration ratio. The SOLARDETOX treatment plant is installed at the Hidrocen factory (Madrid, Spain).
Fendrich et al.
[92] presented solar concentration technologies for wastewater remediation. They concluded that though mostly on model systems, recent results open promising perspectives for using concentrated sunlight as the energy source powering advanced oxidation processes, such as photocatalytic degradation by TiO
2. Additionally, they identified the photocatalyst materials capable of efficiently working with sunlight and the transition to real wastewater investigation as the most critical issues to be addressed by research in the field.
This entry is adapted from the peer-reviewed paper 10.3390/catal13010026