The temperature of the incubation chamber is one of the most important (the incubation chamber temperature is the laboratory static and shaking incubator temperature for the bacteria growth that is kept 37 °C for effective growth of bacteria; Higher temperature may affect the growth of bacteria) operating parameters that might influence bacterial growth and, as a result, the bio-mineralization process
[2]. The catalysis of urea hydrolysis, like other enzymatic reactions, is a temperature-dependent process
[61]. The ideal temperature, however, lies between 20 and 37 °C, depending on the surrounding conditions and the concentrations of chemical reagents in the fermentative media
[62] [63][64]. The rate of urea disintegration is increased by 5 and 10 times, respectively, when the reaction temperature is raised from 10 to 20 °C and between 10-15 °C, according to previous research
[65]. In a solution medium with 0.02 gram/Liter of catalyst, it discovered that increasing the temperature from 20 to 50 °C enhances calcium carbonate production
[66]. However, a relatively high temperature had a negative impact on bacterial metabolism and enzyme function
[44]. Despite a consistent urease activity at comfortable temperature (35 °C), it discovered that at a high temperature (55 °C), the enzyme activity significantly declines
[67].
3. Tests for Assessing the Bio-Mineralized Calcium Carbonate Based SELF-Healing Concrete
Several methods for evaluating the crack self-healing effect were put forth in order to evaluate the potential of microbial self-healing concrete (MSHC) to close cracks. For instance, X-ray diffraction, scanning electron microscopy, and energy dispersive spectroscopy (XRD) [68][69] were employed to examine the shape of crystals that precipitated at the break. The ability of the bio-mineralized self-healing precipitate was further evaluated using water permeability, porosity, and chloride ion permeation resistance [70][71] and nondestructive detection methods including ultrasonic and acoustic emission were used to assess the effectiveness of bio-self-healing. concrete [72][73][74][75]. TEM analysis was used to assess the sealing ability or deposition of MICP by [76] on archeological gypsum plasters employed M. xanthus bacteria. However, characterization techniques like SEM, XRD, and water permeability are only suitable for testing in the lab and not real engineering since they cannot concurrently satisfy the demands of operability and nondestructive testing. Acoustic emission and ultrasonic technology are expected to be heavily used in practical engineering due to the advantages of simple detecting equipment, practical operation, and economical detection. The majority of MSHC research is based on previously published experiments.
However, several scientists have chosen to use measurements of the deposited minerals to confirm the encapsulation of bacteria in self-healing concrete [44][77][78][79][80]. In the realm of encapsulating materials in self-healing concrete, techniques of testing based on the restoration of mechanical characteristics by the transmission of ultrasonic waves are still unexamined. Quantification of precipitated crystals in cementitious and biochar materials has been investigated using TGA for concrete or mortar samples.
Microstructure tests are carried out using tools including scanning electron microscopes (SEMs), field-emission scanning electron microscopes (FESEMs), and X-ray diffraction to identify and describe embedded materials after self-healing (XRD) [81] used bio-cement with a variable urea-CaCl2 and bacterial cell density concentration. Based on its 40% greater compressive strength than conventional concrete, improved material finish, aesthetic qualities, and environmental effect, the authors came to the conclusion that bio-cement has potential as a sustainable design material.
ATRIR [82][83] revealed the chemical composition of the deposit, which consisted of a calcite and aragonite combination in addition to two CaCO3 polymorphs. For more confirmation of the characterization results, the XRD is frequently employed, along with SEM-EDX by various researchers. This method was utilized to search for verified healing agents in the precipitates based on macrostructural and SEM inspection. The most prevalent microstructural test used by investigators to trace deposition products in crack specimens was SEM analysis [84]. In addition, for qualitative and quantitative elemental analysis, several researchers have combined EDS with SEM [85][86][87][88]. X-ray tomography, Raman spectroscopy, and Nuclear Magnet Resonance (NMR) can be utilized to monitor and study crack healing qualitatively and quantitatively besides the existing microstructure level research [89][90]. The nanotechnology level study of self-restoration effectiveness by bacteria encased materials has yet to be completed. These tests are performed to ensure that the results of the microstructural testing are as reliable as possible. Nanoscale tests should be performed to determine the bonding strength within the fractures at the interface between the deposited minerals and the cement substrate [91].
4. Sealing Ability and Recovery of Mechanical and Durability Properties
The crack width healed, the precipitate’s bonding with the mixture and structure, and the precipitate’s strength would all influence the restoration of original concrete properties. For efficient self-healing, properties that are as close to those of the original concrete as possible are desirable. The capability of bio-based self-healing concrete to heal is dependent on a variety of parameters, such as the curing condition, concentrations of doable spores and nutrients, the age of the concrete, and the amount of time it takes for the concrete to heal. Healing can occur in two different formats: calcium carbonate precipitation to close the fractures, and carbon dioxide produced by bacteria metabolism reacting with unreacted portlandite at the crack region to make more deposits. The use of a bio-based restoring chemical was found to cure a wide variety of fracture widths. Although it is dependent on a variety of conditions, healing efficiency is optimal when crack width is kept between 100 and 200 µm.
4.1. Recovery of Mechanical Properties
Wang [92] used glass tubes to encapsulate bacteria cells in PU and silica gel. The crack width used to measure mechanical strength recovery was around 0.35 mm. In the case of silica gel, there was more calcium carbonate precipitation, but only around 5% strength recovery. PU-containing specimens recovered between 50 and 80 percent of their potency. Conversely, the bacterial role in regaining mechanical strength was questioned because the strength recovery for live and dead bacteria cells was not significantly different. The precipitate quantity was larger in silica gel than in PU, although PU had a higher strength recovery. It’s reasonable to assume that PU, as an excellent sealing agent, played a major role in the mechanical strength recovery.
4.2. Recovery of Durability Properties
In concrete, effective self-healing means that the durability and mechanical strength are totally or almost restored to the original specimen. Water permeability and water absorption tests are frequently used to assess durability. Healing cracks also entails sealing any voids or linked pores through which foreign chemicals from the air or water could enter. As a result, water permeability and absorption are reduced. Pore blockage by calcium carbonate, which has a relatively low solubility, causes absolute permeability reduction through bacterial action
[93][94][95].
Wang
[96] used hydrogel as an encapsulation for bacteria spores and bio-reagents and reported a significant reduction in permeability of about 68 percent. Even for 0.3–0.4 mm cracks, the maximum crack size of 0.5 mm was repaired, albeit there was a wide range of healing ratios (40–90%). However, when compared to when only hydrogel was used, there is an improvement. Due to the proportionate dispersion of spores and bio reagents when encased along in hydrogel, better healing may be expected. Because of this encapsulation approach, bacteria would have fast reach to nutrients and precursor compounds in the case of cracking. Furthermore, in addition to bacterial precipitation, some autogenous healing helped by internal hydrogel curing may improve permeability reduction.
5. Field Application of Bio-Mineralized Self-Healing Concrete
To test the capacity of Sporosarcina pasteurii cells to self-heal, broken rock was repaired with the cells
[97]. In 17 h of handling, a sizable amount of calcite precipitated (around 750 gramme), and a water permeability test of a single fracture over a large area revealed a significant reduction. These results suggested that MICP can be utilised to reduce the porosity of cracked rock in practise, suggesting that a MICP-based strategy would be a suitable choice for reducing unwelcome groundwater flow through fractured channels. In another investigation, investigators used bacteria to treat the faces of limestone at various temperatures in attempt to discover the optimal microorganism for practical use
[98]. The limestone was reinforced to depths of 30 mm after a surface modification with bacteria twice in 12 h. The treatment cost was reduced to within the reach of common consolidates by optimizing urease dosages and carbonate precursor solutions. Subsurface drilled well fluid leaking in typical oil and gas exploitation or carbon capture technologies can be stopped by using the MICP a novel way by sealing gaps and porosity and lowering the system’s liquid penetration. So because activation solutions used in MICP-based sealants are water-soluble and have lower viscosities than those used in cement-based sealants, they are simpler to move into the consolidation deposit and are therefore more appropriate for usage with cracked structures. The study used conventional fluid conveyance methods to treat sandstone strata fractures 340.8 m below ground level with a S. pasteurii culture and a urea-calcium mixture (packer and bailer). Leading to decreased in insertion rate of flow from 1.9 to 0.47 L/min and a reduction in well pressure gradient from more than 30 percent to 7 percent. Moreover, following MICP therapy, the crack extension stress while re-fracturing improved in comparison to before MICP administration. The applications for which bio-concrete might be especially advantageous are shown in
Figure 1. These applications’ main goals were to cut maintenance costs and prevent water infiltration. Additional uses included those for hard-to-reach locations, the nuclear sector, water-retaining structures, and airports
[99].
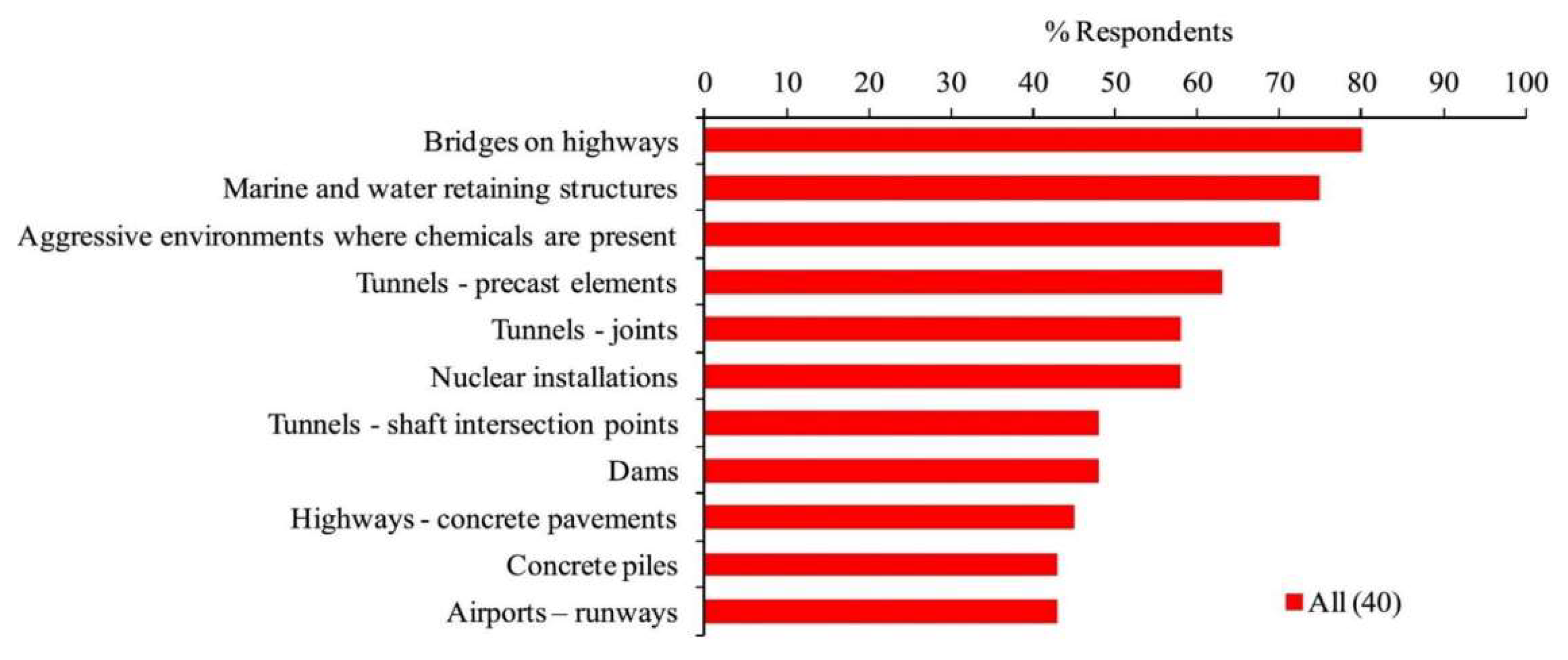
Figure 1. Proposed application areas of self healing bioconcrete
[100].