2. Evolutionary Perspective of PROTACs
Although the concept of the PROTACs has recently emerged, its evolution is such that we can divide it into three generations (Figure 3).
2.1. First Generation—Peptide-Based PROTACs (2001–2008)
The first PROTAC appeared in 2001, in a work led by Crews and Deshaies, whose target was MetAP-2
[18]. Designated as “PROTAC-1”, it covalently binds to the POI via ovalacin (angiogenesis inhibitor) and recruits one E3 ligase through the phosphopeptide, IkBα, with 10 aa
[18]. However, its large size reduces the ability to get inside the cells, having to be micro-injected into them
[18]. On the other hand, its phosphopeptide part makes it susceptible to intracellular phosphatases
[18].
In 2003, the same group, recruiting the same E3 ligase as the previous PROTAC, published an estradiol-based PROTAC, for which the target was the estrogen receptor (ER), α isoform, and a dihydroxytestosterone (DHT)-based PROTAC, for which the target was the androgen receptor (AR)
[19]. Both compounds demonstrated that they could degrade their respective targets quickly and effectively
[19]. These targets are implicated in the progression of breast cancer (BC) and prostate cancer (PC), respectively
[19]. Despite this, the limitations are the same as those of PROTAC-1
[19].
To overcome these obstacles, the Crews group, in 2004, created the first peptide based PROTACs able to permeate cells
[20]. To this end, the new molecules, through a peptide derivative of the hypoxia-inducible factor-1α (HIF1α), recruit the Von Hippel-Lindau (VHL) E3 ligase, and presented a poly-D-arginine chain in the ligand that recruits the enzyme, allowing for an increase in cell permeability and conferring proteolytic resistance. The chosen targets were the AR and FKBP12
[20].
Over the years, new groups began to investigate, and innovative optimizations were performed, which allowed them to obtain new molecules. For example, in 2008, PROTAC-A against AR (ligand: DHT) and PROTAC-B against ER (ligand: estradiol) appeared
[21]. Both PROTACs use the VHL E3 ligase, but the ligand that recruits it results from a shortening of the peptide derivative of HIF1α, becoming a pentapeptide
[21]. Overcoming the difficulties in penetrating cell membranes, the two compounds were able to inhibit cell proliferation, with an increase in activity compared to previous PROTACs
[21].
Overall, although this generation achieved the selective degradation of their targets, the concentrations required for this to happen were in the micromolar range
[18][20][21].
2.2. Second Generation—Small Molecule-Based PROTACs
Given that the PROTACs, until the present date, were of peptide origin and had high molecular weights, their stability and cellular permeability were their weak points, forcing the creation of new strategies to obtain better results.
Therefore, in 2008, the Crews group presented the first and revolutionary “small molecule-based PROTAC”, which started a whole new generation of PROTACs
[6].
This first small molecule-based PROTAC consisted of a non-steroidal AR ligand, and a mouse double minute 2 (MDM2) E3 ligase recruiter, nutlin-3a, connected by a linker
[6]. The fact that the ligands chosen in PROTACs are small chemical molecules, substantially reducing their molecular weight, promotes their entry into cancer cells
[6]. However, concentrations in the micromolar range are still required
[6].
In the years that followed, new targets, ligands, and E3 ligases were chosen for the design of the new PROTACs with the aim of increasing cellular activity, reducing the concentration required for the degradation, and increasing stability, among many other aspects.
With remarkable success, small-based PROTACs have emerged to recruit other E3 ligases besides MDM2 (cellular inhibitor of apoptosis protein 1 (IAP)
[22]; cereblon (CRBN)
[23]; Von Hipple-Lindau (VHL)
[24]).
Over the last few years, new PROTACs have emerged, presenting increasingly better features in terms of allowing oral administration, good cell permeability and solubility, as well as a long duration of action and efficacy at nanomolar concentrations, making them an excellent alternative to the disadvantages presented by conventional therapies, such as SMIs or mAbs
[5][14][25].
2.3. Third Generation—Spatiotemporal Controllable PROTACs
This new generation of PROTACs results from the application of modern technologies to obtain an improvement in specificity, minimizing the possible cytotoxic and off-target effects as much as possible, ensuring greater safety associated with the treatment using these molecules, and an increase in efficacy and duration of action, or to improve their pharmacokinetic aspects
[5][14][25].
One of the first examples is the phosphoPROTAC, created by the Crews group in 2013, in which a peptide sequence is incorporated, which is phosphorylated only by a particular type of tyrosine kinase, allowing for its activation, when phosphorylated, only in cells that contain this enzyme, with a consequent increase in specificity
[26].
With the objective of obtaining a controlled protein degradation, opto-PROTACs appeared; these have a photolabile caging group, nitroveratryloxycarbonyl, which is a group that blocks the action of the PROTAC
[27]. However, when the molecule undergoes irradiation by a certain type of radiation, it loses this labile group, activating the PROTAC and promoting the degradation of the POI
[27].
With a slightly similar mechanism, the PHOTACs emerged, which, when irradiated by a certain type of radiation, change their conformation, going from a state of inactivation to activation
[28] or vice-versa
[29].
Both technologies make it possible to have temporal and spatial control over the action of the PROTACs, thus, ensuring that they act in the right place, at the right time, and in the right concentration, increasing their safety and efficacy
[28][29].
The so-called in-cell click-formed proteolysis-targeting chimeras (CLIPTACs) allow for better bioavailability because this strategy consists of dividing the PROTAC into two units which are joined at the site of action, promoting the ubiquitination of the POI
[30].
More recently, new ideas have emerged, such as conjugating antibodies with PROTACs, and forming the antibody-PROTAC conjugate (APC) for the treatment of cancer in order to allow for the delivery of the drug to the cancer cells in a specific way
[31].
3. PROTACs That Recruit MDM2 E3 Ligase
Although presenting very promising results, the first generation of PROTACs, regarding the degradation of key proteins in tumor development
[32][20], has some disadvantages, mainly due to high molecular weight, which ends up compromising its stability and cellular permeability, such as hindering the entire process of synthesis and purification
[6]. These aspects are extremely relevant when the aim is to develop a drug since a complex synthesis and purification process causes disadvantages for the pharmaceutical industry and, thus, compromises its entry into the market
[33].
The creation of smaller PROTACs, using small chemical ligands, as well as linkers of a chemical origin, is a way to circumvent the problems presented by the previous generation, as was seen with the creation of the first small-based PROTAC in 2008, which, interestingly, was also the first MDM2-based PROTAC
[6].
When this first MDM2-based PROTAC was created, the main advantage revealed at the time was that it only allowed for a reduction in its dimensions
[6]. However, recruiting the MDM2 E3 ligase brings more benefits in terms of cancer treatment since it is responsible for the regulation of the tumor suppressor p53, which has a relevant role in the development of a high number of cancers
[34][35].
Although the number of PROTACs recruiting MDM2 is still reduced, they have enormous potential in certain types of cancer, with a focus on those in which both the POI and E3 ligase are overexpressed
[36]. This is because an MDM2-based PROTAC, in addition to having the ability to promote POI degradation by recruiting MDM2 with this binding ends up preventing its main function, that is, it binds to p53, leads to an increase in intracellular levels of the suppressor (
Figure 3)
[16].
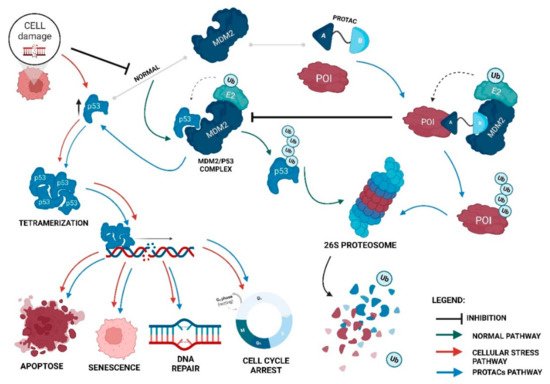
Figure 3. The impact of MDM2-based PROTACs on the MDM2/p53 cycle. Under normal conditions, p53 levels are kept low due to the presence of the MDM2 E3 ligase, which, when binding to the tumor suppressor, promotes its polyubiquitination, being subsequently recognized by the 26S proteasome, and degraded. When cell damage occurs, p53 is stabilized and forms a tetramer, and its ubiquitination by MDM2 is prevented. The tetramer is transported towards the nucleus, where it activates the transcription of those genes responsible for the processes of apoptosis, senescence, DNA repair, and cell-cycle arrest. However, in the presence of an MDM2-based PROTAC, when recruiting MDM2, this prevents it from binding to its natural substrate, p53, increasing the levels of the suppressor, which culminates not only in the degradation of POI, but also in the activation of processes that prevent the development of cancer cells
[16].
Therefore, with MDM2-based PROTACs, it is possible not only to degrade the target oncogenic proteins, but also prevents MDM2 from binding to p53, activating its tumor suppressor action
[16]. This duality of effect presented by this type of PROTAC makes their use in cancer therapy of great interest; they may become a great therapeutic weapon in the coming years.
MDM2-based PROTACs - Summary table of all MDM2-based PROTACs created to date for the treatment of several types of cancer:
https://www.mdpi.com/1841390
4. Advantages of MDM2-Based PROTACs
Nowadays, conventional therapies with mAbs, capable of blocking extracellular targets or SMIs which can inhibit extra and intracellular compounds (and that respect Lipinski’s Rule of 5 (Ro5) for oral administration), unfortunately have some limitations
[37].
However, SMIs perform a stoichiometric inhibition
[38], in which one molecule of inhibitor inhibits one molecule of the target, following an occupancy-driven model, in which high concentrations are required to maintain a certain level of occupancy of the target to exert the desired effect
[38][39]. Consequently, the use of high concentrations promotes the occurrence of adverse effects associated with some disadvantages, such as the inability to inhibit “undruggable proteins”, protein accumulation, and increasing its expression or the development of resistance
[39] [37].
The development of PROTACs, more specifically MDM2-based PROTACs, can promote therapeutic effects through target degradation, as seen above, and has some advantages over inhibition, such as the following.
-
PROTACs follow the event-driven model—a molecule of PROTAC can degrade several molecules of POI. Thus, this presents a catalytic cycle of degradation, allowing for its use with substoichiometric concentrations
[40][41];
-
PROTACs can display cooperativity—binding to a second protein in PROTACs can lead to the formation of the ternary complex being favored (positive cooperativity), or it can lead to the formation of binary complexes (negative cooperativity) or simply remain not affected (neutral cooperativity). If positive cooperativity exists, a stable ternary complex can be formed even in the presence of low affinities with the POI
[38][39];
-
Fast, selective, and sustained POI degradation—selective degradation, even for the isoforms of the target that may not have an active site, (with the use of low concentrations (µM—nM)) is associated with a reduction in the activation of the respective signaling pathways
[40][42][43][43];
-
Ability to cause cellular apoptosis, delay the cell cycle, decrease proliferation and metastasis of tumor cells—the activation of the p53 suppressor pathway in vitro, ex vivo, and in vivo
[40][42][43][43];
-
PROTACs do not cause a compensatory expression of POI, not even intracellular accumulation—there are no changes in the mRNA expression levels of the respective target protein
[42][43][43];
-
PROTACs are less susceptible to the development of resistance—the resistance me-chanism will have to encompass both of the pathways of the PROTAC, given that, in the absence of the degradation capacity, the inhibition of the target or MDM2 by itself may still have anticancer action
[42].
5. Disadvantages of MDM2-Based PROTACs
For a PROTAC to be able to exert its action, it must go inside the cell, and this can be a limiting step given that they are usually large molecules
[41]. As seen above, the strategy of reducing the size of the ligands or adding peptides that promote membrane permeability (poly-arginine tail) can help to overcome these difficulties
[41].
Furthermore, PROTACs, when in high concentrations, can develop the hook effect
[42]. However, if this presents positive cooperativity, then this effect can be attenuated, favoring the formation of the ternary complex and reducing the required concentrations
[44].
From a pharmacokinetic viewpoint, PROTACs are usually given by parenteral administration
[37]. Although not following Lipinski’s Ro5, given that MDM2-based PROTACs have the highest molecular weight and most lipophilic type of PROTACs, with 3 aromatic rings in the MDM2 ligand, their oral administration will be a challenge, but, currently, there are examples of PROTACs that may have good bioavailability when administered orally, and MDM2-based PROTACs may become good candidates for oral anticancer therapies
[45]. In addition to absorption issues, its metabolism is also an aspect to be taken into account since, in vivo, they will give rise to metabolites, which, even if they are not able to degrade the target, may perhaps inhibit POI or MDM2
[45]. Furthermore, the fact that these PROTACs have a high molecular weight may hinder their ability to enter the interior of liver cells and, thus, be metabolized; in this sense, studies to assess this ability and predict its impact are necessary
[46].
Another relevant issue is related to the fact that genotoxic stress promotes the expression of MDM2 isoforms that lack the full N-terminal p53 binding domain and varying extensions of the central acid domain, resulting from alternative splicing, with the consequential loss of its ubiquitinating activity
[47]. However, as far as we know, there are no studies that allow us to understand the impact that these isoforms may have on the performance of MDM2-based PROTACs
[47].
Therefore, MDM2-based PROTACs offer a full set of aspects that can be improved, making it necessary to conduct more studies to clarify, create, and improve these promising molecules in therapy.
Conclusions
The MDM2-based PROTACs, although still under-represented within the universe of PROTACs, have unique and very advantageous anti-tumor characteristics, given that, in addition to degrading proteins, such as the AR
[6], BCR4
[42], PARP1
[48], or their own MDM2
[40], they have the ability to activate the p53 tumor suppressor pathway. This leads to the consequent activation of apoptosis processes in tumor cells, leading to their cell death, presenting, in the end, a synergistic effect—an effect much superior to the use of isolated inhibitors
[42]. Therefore, with a dual mechanism of action, which requires the formation of a stable ternary complex, MDM2-based PROTACs, with some refinement, may well become candidates for the future anticancer therapeutic arsenal, as they have already proven in certain cases to be able to be effective in vitro and in vivo at concentrations in the nanomolar order, given their catalytic and synergistic mode of action, and with characteristics that overcome the current disadvantages of conventional therapies, highlighting the lack of selectivity for the target and cell type, the development of resistance, tolerance, accumulation of the target protein, and processes of up-regulation of the target
[40][42][43][43].
Furthermore, the great advantage of PROTACs, and of those that recruit MDM2, lies in the possibility of degrading previously “undruggable proteins”, that is, they allow for the degradation of targets which do not have an active site, thus being able, with these new molecules, to interfere with new signaling pathways crucial for the development and proliferation of cancer cells, something that has never been explored before
[7].
Therefore, the MDM2-based PROTACs need more studies to be improved, present new characteristics, as well as overcoming their current limitations. However, everything points to these molecules having an incredible potential to become an innovative therapeutic strategy for cancer treatment.