Modern humanity wades daily through various radiations, resulting in frequent exposure and causing potentially important biological effects. Among them, the brain is the organ most sensitive to electromagnetic radiation (EMR) exposure.
1. Introduction
Microwaves are recognized as nonionizing radiation, with a broad frequency spectrum ranging from 300 MHz to 300 GHz. Specifically, the bands from 300 MHz to 3 GHz are UHF (ultra-high-frequency), 3–30 GHz are SHF (super-high-frequency), and those from 30 to 300 GHz are EHF (extremely high-frequency). Additionally, microwaves that exceed a peak power of 100 MW with an operation frequency lying between 1 to 300 GHz are generally considered high-power microwaves (HPMs). HPMs are commonly employed in modern technologies and have been proven to be indispensable in our lives, with uses in the commercial, military, and medical fields
[1][2][3][4]. Pulse microwave sources and non-pulse microwave sources are the two types of HPM sources available. A microwave source with a rising edge of sub-nanoseconds or picoseconds is referred to as a pulsed microwave source. A primary drive (explosive or pulse generation system), a pulse compression system, a microwave generation source, and an antenna are all included. Through low-speed storage and fast release of energy, pulse sources typically transform energy into short-pulse EMR. Various HPM sources exist to meet the demand for HPM in applications, with the majority of them undergoing research to increase conversion efficiency. The generation of HPMs also represents an active area of research
[5][6][7][8][9][10][11][12][13][14][15]. HPM has arisen as a new technology that provides a wide range of original applications while also providing innovative methods and changes to existing technologies from previous decades. HPMs can be used for a variety of future applications and to improve the performance of existing technologies in our daily lives. Further, progress in radio-frequency microwaves, among others, has provided novel diagnostic and therapeutic methods; whereas microwaves between 400 kHz to 10 GHz are presently being studied for their therapeutic purposes in the medical field
[16][17][18], and have been explored for diagnostic applications, such as early-stage cancer and tumor detection, organ imaging, etc.
[18][19][20][21][22][23][24][25][26][27].
Radio waves are electromagnetic waves that range from 3 kHz to 300 GHz in frequency. To observe astronomical objects, radio waves are commonly employed as envelope signals on radio communication and wavelength channels. Microwaves are a form of short-frequency radio waves. They can be categorized as a radio wave subclass. Microwaves have a frequency range of 300 MHz to 300 GHz. Microwaves are commonly employed in microwave ovens because the resonance frequency of water molecules is in the microwave range. Radars, astronomy, navigation, and spectroscopy all employ microwaves. Accordingly, the development of advanced electronics and novel microwave-based systems has made microwaves an important part of our daily lives. More specifically, the increasing number of radio-wave-based applications has led to the investigation of their biological effects.
2. Interactions of Biological Systems with Electromagnetic Radiation (EMR)
Owing to the existence of various types of radiation in our daily living environments, the effects of biological system exposure represent an important area of research and include both the biological effects and safety levels of these types of radiation. Several studies from in vitro and in vivo research have indicated that these radiations directly affect (positive or negative) biological systems.
Figure 1 shows the various sources of EMR with their corresponding frequency ranges, whereas
Figure 2 illustrates the various alterations caused by EMR being transmitted into the human body, where these effects can be detrimental, useful, or neutral. This research topic has received significant interest in the last few decades. Specifically, EMR energy absorption by the human body, specifically in the head and neck, has received increased attention recently
[28]. Indeed, numerous studies have been conducted on the subject of defining EMR interactions in biological systems
[29][30][31]. Notably, all biological levels are affected by EMR, including microbes, animals, and humans
[32]. The physiological alterations to EMR are dependent upon the operating frequencies and peak power. The in-depth mechanisms of microwave bio-interactions have shown that EMR acts as a helping agent to induce genetic changes in biosystems
[32]. When studying microwave absorption in animals and humans, various uncertainties remain regarding the relative contributions of indirect heat effects, as well as direct non-thermal interactions to physiological alterations. The biological interactions that occur at the microscopic level are correlated with the dielectric properties of biomacromolecules and bulky molecular units (i.e., cell-membrane receptors and enzyme complexes)
[30]. Most cell types contain an electrical gradient (membrane potential) of ~0.1 V across the
40 A°
width of the double layer of fat molecules, typically useful for providing an essential structure of a cell membrane
[33]. Further, this electrical gradient (105 V·cm
−1) is believed to be an effective wall against cell stimulation caused by weaker EMRs
[33].
Figure 1. Depiction of daily commercial and household radiation sources with corresponding operational frequency ranges.
Figure 2. A generalized representation of the interactions between EMR and biological systems, with common effects. The EMR has an impact on molecular ions and electrons, as well as ROS, protein, and DNA/RNA levels. Furthermore, the EMR has cytotoxic effects on cells by causing degeneration, apoptosis, and necrosis. EMR has a strong impact on the central nervous system, reproductive system, cardiovascular system, and hematological system. Furthermore, the constant and long-term exposure of EMR to a biological system raises tissue temperature, which is a frequent effect of different stimuli.
3. Possible Biological Effects and Mechanisms of EMR
The precise mechanisms by which microwaves affect the biological system remain largely unknown due to different equipment and inadequate techniques, bringing uncertainty into the available data. Despite this, many hypotheses have been generated to describe the possible mechanisms of the biological effects of microwaves
[34]. An overview of these mechanisms is shown in
Figure 2, whereas the most common mechanism is shown in
Figure 3. Specifically, microwaves cause electrons or ions to vibrate owing to resonance, which then collides with other molecules inside biological tissues. Opposite charges swing to opposite sides, and charge polarization occurs in the presence of the electric field (EF) provided by the microwave. This polarization is applicable not only for free charges that are irregularly present in the biological tissue but within individual particles for which the net electric charge is zero as well. Accordingly, this polarization induces the formation of electric dipoles
[33]. Continuous repolarization of the induced dipoles and an energy-consuming phenomenon that absorbs the EF energy occurs with the alternating EF. Biological tissues contain large amounts of water
[33]. In an alternating EF, the dipoles continually oscillate around their axes, helping to absorb electric energy
[35]. Several theories describe the effects of EMR in biological systems
[35][36], and an overview is provided in
Figure 2. The emission of continuous radio waves (e.g., microwaves) increases the temperatures of the living tissues, whereas nonionizing EMR may also cause biochemical changes that lead to various effects (good, bad, and neutral). Indeed, it is possible that all possible mechanisms depend on resonance, coherence, and CNS functioning, as well as the stimulation of muscles, reactive oxygen species (ROS), proteins, DNA, and RNA (
Figure 2). The input of energy through an external signal may be concentrated when the EMR wavelengths are equal to the molecular energy level differences, resulting in an upsurge in signal strength. Most ions are attached to water, and the dispersion of energy increases system loss when acting on water particles with radio frequencies obtained in resonance. A common concept used to understand the effects of EMR on cells is the induction of supplementary potentials on cellular membranes to interfere with ionic transport
[35]. Such changes are only possible when external fields are sufficiently strong, significantly higher than the voltages generated by mitochondrial membranes. Exposure to non-physiological voltage in cell organelles has shown that when the membranes are wider than the cellular membrane, and organelles are comprised of large ionic concentrations, more EMR energy is transmitted through the organelle membrane
[35]. This mechanism is used to understand the effects of EMR on cells by inducing variations in molecular bonds that can impact protein enzyme activity
[37]. Notably, cellular proteins have diverse structures, and the effects of EMR exposure can vary accordingly
[37]. Earlier studies found that protein denaturation, aggregation, and stability can be affected by EMR
[38][39], which is why the enzymatic efficiency of a protein is also structure-dependent. Few amino acid side chains in proteins are known to be polar and respond differently when exposed to different EMR. Microwaves sensitively affect some biological organs in addition to the CNS, reproductive, cardiovascular, and hematopoietic systems. Furthermore, EMR exposure increases reactive oxygen species (ROS) levels inside tissues
[40], leading to macromolecular changes, including DNA/RNA and proteins. This induced oxidative stress increases malondialdehyde, leading to membrane lipid injury, and a reduced glutathione concentration, which plays a key defensive role against various diseases
[41].
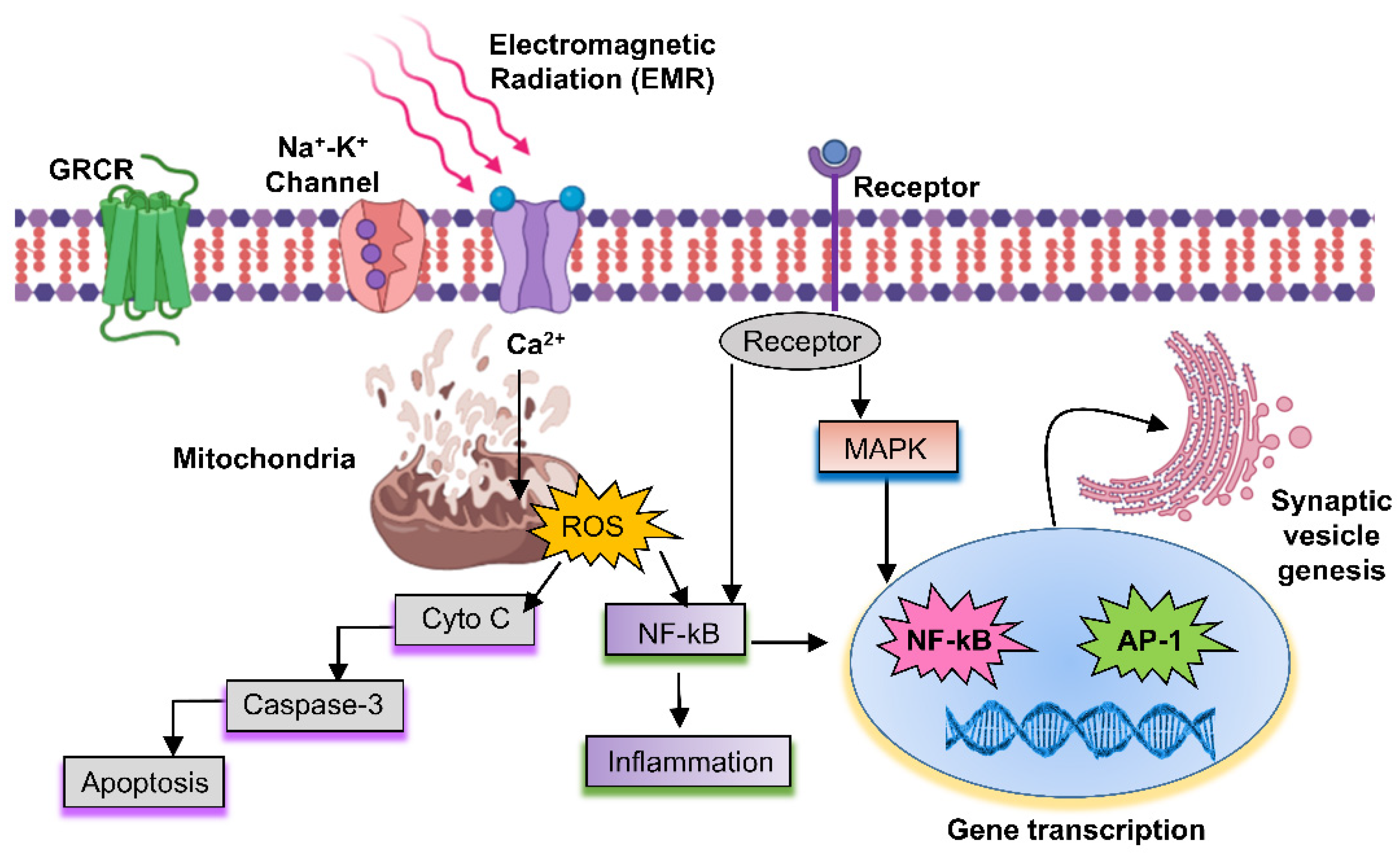
This entry is adapted from the peer-reviewed paper 10.3390/ijms23169288