The discovery of ferrocene is often associated with the rapid growth of organometallic chemistry. Dendrimers are highly branched macromolecules that can be functionalized at will at all levels of their structure. The functionalization of dendrimers with ferrocene derivatives can be carried out easily as terminal functions on the surface, but also at the core, or at one or several layers inside the structure. Depending on the desired location of the ferrocenes in the structure of phosphorhydrazone dendrimers, the ferrocenes should be functionalized differently. For the grafting to the surface, the ferrocene should bear a phenol group, suitable to react in substitution reactions with the P(S)Cl2 terminal groups of the dendrimers. To be used as core, the ferrocene should have two aldehyde functions, from which the synthesis of the dendrimer will be carried out. To be introduced in the branches, at all layers or within a single layer, the ferrocene should replace hydroxybenzaldehyde; thus, it should bear both a phenol and an aldehyde.
1. Introduction
Thousands of publications concern ferrocene derivatives, and their discovery represented a turning point in organometallic chemistry, as the leading member of the metallocene family. Ferrocene consists of two cyclopentadienyl rings bound on opposite sides of a central Fe2+ atom. It has been shown to have excellent thermal and photochemical stability, and to undergo a facile and reversible one-electron oxidation to ferrocenium cation. The functionalization of one or both cyclopentadienyl units has led to their grafting to numerous types of molecules, and to polymers and to dendrimers. Dendrimers are a very special class of polymers, constituted of repetitive branching units but not synthesized by polymerization reactions. Their step-by-step synthesis ensures a perfectly defined and highly reproducible structure [1]. Among the diverse types of dendrimers, phosphorhydrazone dendrimers, which possess one phosphorus atom at each branching point [2], occupy a special place among “inorganic” dendrimers [3] thanks to their easy and versatile functionalization, in particular on their periphery [4], and to the numerous properties that have been already demonstrated with them. Figure 1 displays the chemical structure of phosphorhydrazone dendrimers of generation 2, built either from P(S)Cl3 (1-G2) or cyclotriphosphazene N3P3Cl6 (2-G2) [5].
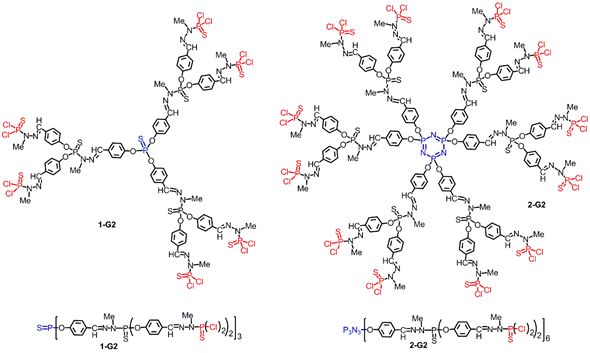
Figure 1. Structure of second generation phosphorhydrazone dendrimers. Left: built from P(S)Cl3 as core; right: built from N3P3Cl6 as core. Upper part: full structure; lower part: linear structures with parentheses to draw the same dendrimers.
Depending on the desired location of the ferrocenes in the structure of phosphorhydrazone dendrimers, the ferrocenes should be functionalized differently.
2. Ferrocenes on the Periphery of Dendrimers
Figure 2. Ferrocenylphenols that have been linked to the surface of phosphorhydrazone dendrimers.
Figure 3. Synthesis of ferrocenes 4, 5, 6, and 7, starting from the common precursor ferrocene carboxaldehyde.
The next step in way A is the reaction of 4-lithioanisole obtained by transmetalation of 4-bromoanisole by n-butylithium on the aldehyde, followed by deoxygenation with sodium cyanoborohydride in presence of titanium tetrachloride. Finally, the methoxy group is transformed to hydroxyl group by reaction with boron bromide, to provide the ferrocene 4, after 9 steps from ferrocene carboxaldehyde [11]. The ferrocene derivative functionalized with both an aldehyde and an iodine (way B) is reacted in a Suzuki coupling reaction with 4-methoxyphenylboronic acid to yield enantiomerically pure 4-methoxyphenyl group on the 2 position of ferrocenecarboxaldehyde. The aldehyde is then converted to a methyl group using sodium cyanoborohydride in presence of titanium tetrachloride Finally, the methoxy group is deprotected to provide compound 5 after 9 steps, as was obtained ferrocene 4 [11]. The (S)-2-(diphenylphosphino) ferrocene carboxaldehyde obtained in way C is the precursor of both ferrocenes 6 (way C1) and 7 (way C2). In way C1, 4-lithioanisole is first reacted on the aldehyde; then, the phosphine is protected with BH3. Reactions with sodium cyanoborohydride in presence of titanium tetrachloride and then with boron bromide are carried out as in way A, to provide compound 6-BH3. The phosphine is finally deprotected using DABCO (1,4-diazabicyclo[2.2.2]octane) to provide the ferrocene 6 after 11 steps [11]. In way C2, the aldehyde is reduced to alcohol and the phosphine is oxidized to thiophosphine using sulfur. In a second step, 4-hydroxythiophenol is reacted on the alcohol, providing directly a phenol. The last step is the reduction of the thiophosphine with tris (dimethylamino)phosphine, providing the ferrocene 7 in 9 steps (Figure 3) [12].
The ferrocenes functionalized on both cyclopentadienyl rings (compounds
8,
9, and
10) are easier to synthesize, as shown in
Figure 4. The common precursor in these cases is 1,1’-dibromoferrocene, on which one bromine is treated with 4-bromoanisole in a Negishi cross-coupling reaction. The direct grafting of diphenylphosphine on the other cyclopentadienyl ring is carried out by lithiating the ferrocene, then quenching with diphenylchlorophosphine. The deprotection of the phenol using tetrabutylammonium fluoride provides the ferrocene
8 after only three steps (
Figure 4)
[13]. A slightly different procedure is used for introducing a linker between the ferrocene and the phosphine (compounds
9 and
10)
[15]. The lithiated ferrocene in presence of ZnCl
2 and [Pd(PPh
3)
4] is treated with either 4-bromophenyl-diphenylphosphane sulfide or 4’-bromobiphenyl diphenylphosphane sulfide. The corresponding phenol is obtained as previously using tetrabutylammonium fluoride. The last step is the deprotection of the thiophosphine, using Raney nickel, to provide ferrocenes
9 or
10 in only four steps, depending on the linker used (
Figure 4)
[13].
Figure 4. Synthesis of ferrocenes 8, 9, and 10, starting from the common precursor 1,1’-dibromoferrocene.
3. Ferrocene at the Core of Dendrimers
Ferrocene derivatives usable as core should have at least two identical functions. The commercially available 1,1’-ferrocene dicarboxaldehyde 11 was used as core to grow the dendrimer up to generation 4 (11-G4, 64 aldehyde terminal functions) (Figure 5) [10].
Figure 5. 1,1’-ferrocene dicarboxaldehyde used as core of dendrimer 11-G4.
4. Ferrocenes as Branches of Dendrimers at one or Several Layers
The functionalization of ferrocenes usable as branches of phosphorhydrazone dendrimers necessitates one to have a phenol on one side and an aldehyde on the other side. Several ferrocene derivatives have been synthesized bearing both suitable functions, and have been used either at several layers of small dendrimers (ferrocenes 12 and 13, Figure 6) or at a single layer inside the structure of large dendrimers (ferrocenes 14 and 15, Figure 6). The methods of synthesis are relatively similar to those shown in Figure 3. In particular, the ferrocene 14 is obtained by transformation of the methoxy to hydroxyl group in the final step, after seven steps shown in Figure 3 [11]. The main difference between the reactions in Figure 3 and Figure 6 concerns the reaction of ferrocene carboxaldehyde with the lithium salt of N-methylpiperazine, which, after additional reaction with t-BuLi, generates the lithation of the other cyclopentadienyl ring [6]. Reaction with electrophiles such as ICH2CH2I or Bu3SnCl are then carried out. The iodoferrocene is first reacted in a Suzuki coupling reaction with 4-methoxyphenylboronic acid, and then the methoxy group is deprotected with BBr3 to provide the ferrocene 12 [10]. The stannyl derivative is first reacted on the aldehyde with 4-lithioanisole, and then the alcohol is reduced with BH3,SMe2. The tributylstannyl group is converted to an aldehyde using butyl lithium, dimethylformamide, and then water. The final step for the synthesis of compound 15 is the cleavage of the methoxy group to provide the phenol, as done previously for compound 12.
Figure 6. Methods of synthesis of ferrocenes functionalized by both a phenol and an aldehyde, which are suitable to be used as branches inside dendrimers. The seven steps for the synthesis of 14 are shown in Figure 3.
Ferrocene 13 is obtained in a different way, as the starting compound for its synthesis is the 1,1’-ferrocene dicarboxaldehyde. A first aldehyde is protected with ethylene glycol, then the second aldehyde is reacted with 4-methoxybenzyltriphenylphosphonium chloride in the presence of n-BuLi to yield a mixture of diastereoisomer (E/Z = 1) by a Wittig reaction. Reaction with iodine in refluxing toluene followed by an acidic hydrolysis provides a complete conversion to the E-isomer of the corresponding aldehyde. The last step to get ferrocene 13 is the deprotection of the methoxy group to hydroxyl, as carried out for many other ferrocenes shown in this review.
4.1. Ferrocenes at all Layers of Dendrimers
The bifunctional ferrocene 12 was first reacted with the P(S)Cl3 core; then, it was used alternately with the phosphorhydrazide H2NNMeP(S)Cl2, up to the second generation 12-G2 (21 ferrocene derivatives and 12 aldehyde terminal groups) (Figure 7) [10].
Figure 7. Bifunctional ferrocene 12 used at all layers of dendrimer 12-G2.
The same process was applied to the other bifunctional ferrocene
13, and was carried out up to the first generation
13-G1 (9 ferrocene derivatives and 6 aldehyde terminal functions) (
Figure 8)
[16].
Figure 8. Bifunctional ferrocene 13 used at all layers of dendrimer 13-G1.
4.2. Ferrocenes at a Single Layer Inside Dendrimers
The chiral ferrocene
14 was introduced at a single layer in phosphorhydrazone dendrimers. It was used first on the surface of dendrimer
1-G3, providing dendrimer
14-G3 [11], then the growing of the dendritic shell was carried out to obtain
14-G3+1, then
14-G3+2, as illustrated in
Figure 9 (24 ferrocenyl groups for all). The same type of experiment was carried out starting from
1-G5 and
1-G9, providing
14-G5+2 (96 ferrocenyl groups) and
14-G9+2 (1536 ferrocenyl groups), respectively
[17].
Figure 9. Bifunctional ferrocene 14 used at a single layer of dendrimer, and dendrimer 14-G3+2.
Other dendrimers bearing a single layer of ferrocenes were also obtained, starting from the dendrimer
2-G2, onto which 24 ferrocenyl derivatives
15 were grafted. The growing of the dendrimer was continued in order to get water-soluble ferrocenyl dendrimers. Ammonium derivatives have been linked to the surface, providing the positively charged dendrimer
15-G3+, whereas carboxylates have been used to provide the negatively charged dendrimer
15-G3- (
Figure 10)
[18].
Figure 10. Bifunctional ferrocene 15 used at a single layer of water-soluble dendrimers 15-G3+ and 15-G3−.
In conclusion, the marriage between dendrimers and ferrocene derivatives is indeed fruitful, with each component providing its specificities. The presence of ferrocenes inside the structure of dendrimers induces a detrimental influence on the electrochemical properties, as the ferrocene's burial in the structure increases. On the contrary, the presence of ferrocenes on the surface of dendrimers provides clean electrochemical data, even for very high generations, such as the eleventh generation. The presence of a phosphine in close proximity of the ferrocene on the surface provides additional properties after the complexation with a metal, in particular for carrying catalysis experiments. Several examples have been already proposed, such as the complexation of palladium for catalyzing asymmetric allylic substitution reaction, and the complexation of ruthenium for the catalytic isomerization of the allylic alcohol 1-octen-3-ol to 3-octanone. In the latter case, the oxidation of the ferrocene induces a strong decrease of the catalytic properties, which are recovered upon reduction of the ferrocenium to ferrocene. This is the very first example of a switch ON/OFF/ON of a dendritic catalyst. Work is in progress to expand the scope of the catalytic properties of ferrocenyl dendrimers, as shown by a recent example with P‑chiral dendritic ferrocenyl phosphine complexes
[19].
This entry is adapted from the peer-reviewed paper 10.3390/molecules25030447