3.1. Competition Dialysis Assay with 1–9
Competition dialysis assay is a potent and valuable quantitative tool for examining compounds of interest that recognize selective structure/sequence of nucleic acid. In this method, an array of nucleic acid sequences and structures is used. Each is placed in a separate MINI dialysis unit fixed in a flotation dialysis supporter inside a glass container and dialyzed against a ligand solution. The free ligand solution is the same for all the structures, but when equilibrium is reached, each of the structure will bind the ligand according to its binding affinity
[54][63].
Nine previously synthesized
[59][60][61] benzothiazole compounds with structural changes (
Figure 2) in position 2 of the imidazole-based benzothiazole core were used to determine the effect of these modifications on the ability of compounds to interact with different DNA and RNA structures.
Figure 2. Summary of competition dialysis results with eight compounds binding to 13 nucleic acid structures and sequences (cbound = concentration of ligand bound to each nucleic acid in µM); sodium cacodylate buffer, I = 0.05 mol dm−3, pH = 7, and +1 mM EDTA.
However, the interaction of eight compounds with 13 different nucleic acid structures was studied (Figure 2): single-stranded and double-stranded polynucleotides, DNA:RNA hybrids, and DNA and RNA triplexes. The interactions of compound 9 with polynucleotides were not further characterized, as compound 9 aggregated in the aqueous solution during the experiment. The interaction of compounds with double-stranded polynucleotides depended on the base composition and secondary structure. Mostly, compounds demonstrated strong binding to DNA triplex ATT. Especially for 5, and even more so, 6, where binding with ATT triplex was stronger than with the double-stranded DNA, RNA, and DNA:RNA hybrids. As the amount of ligand bound to each nucleic acid structure (Cbound) is directly proportional to the ligand binding affinity, it is clear that 1 and 6 displayed the highest affinities toward the majority of the nucleic acid structures. Regarding DNA:RNA hybrids, 6 demonstrated the strongest binding to poly dA−poly rU, while 1 bound slightly better to poly rA−poly dT than to poly dA−poly rU. Only 1 demonstrated preferential binding to single-stranded poly dT, while both 1 and 6 exhibited stronger binding to poly rA. Two metrics were used, the specificity sum, SS and the ratio Cmax/SS, to gain information about the structural selectivity and compound affinity. To calculate the specificity sum, the binding data first need to be normalized relative to the maximal amount bound (Cmax) to any of the structures in this assay. Then normalized amounts for each nucleic acid structure in the assay were simply summed. As 13 nucleic acid structures were used in this experiment, the SS ratio can range from 1, which denotes the binding to only one nucleic acid structure, to 13, which means an equal binding to all structures. According to Figure 3, the best structural selectivity demonstrated 5 and 6. Values for the specificity sum for all studied compounds are shown in Figure 3.
Figure 3. Ratio cmax/SS (A) and specific sum SS (B) for 8 benzothiazole compounds.
Ratio C
max/SS refers to both affinity and selectivity. This ratio is directly proportional to binding affinity. Thus, if C
max is large (high binding affinity) and SS is small (high selectivity), a high value of C
max/SS will be obtained and vice versa
[54][63]. Identification of compounds with the best-combined selectivity and affinity can be obtained by comparison of SS and C
max/SS values. Based on these metrics,
5 and
6 were identified as compounds with the greatest combined selectivity and affinity. Despite the largest SS value,
1 was also selected for further characterization with polynucleotides, as it demonstrated relatively higher C
bound values (amount of ligand bound to each nucleic acid structure), determined for ds- and triplex polynucleotides than other compounds. All three compounds demonstrated selectivity for ATT triplex. Additionally,
6 exhibited the highest affinity toward poly dA−poly rU, while
1 displayed a higher affinity toward poly rA–poly dT.
3.2. Fluorescence Spectroscopy and Isothermal Titration Calorimetry
Isothermal titration calorimetry and fluorescence spectroscopy have been used to characterize the ligand binding to nucleic acid structures
[64][65]. According to the results of the competition dialysis assay (affinities and selectivities), several complexes were selected for detailed characterization:
1-ATT (
Figure 4),
1-poly rA−poly dT,
6-ATT,
6-poly dA−poly rU, and
5-ATT (
Table 2).
Figure 4. (a) Raw titration data from the single injection of 1 into a solution of ATT triplex; (b) ITC experiment of ATT triplex titrated with 1; experimental data (■) and calculated fit (–) for model of two sets of sites. [ATT] = 3.0 × 10−5 M; pH = 7.0, Na-cacodylate buffer, I = 0.05 mol dm−3 + 1 mM EDTA.
Table 2. Data parameters obtained during nonlinear regression (model: one and two sets of sites) for ITC titration for 1-ATT, 1-poly rA−poly dT complex. Binding constants (logKs) a and ratios n a ([bound compound]/[polynucleotide phosphate]) calculated from the fluorescence titrations for ligand-nucleic acid complex d.
Complex |
n1/n2 |
log Ks1/Ks2 |
ΔrH1,2°/kJ mol−1 |
TΔr1,2S°/kJ mol−1 |
ΔrG1,2°/kJ mol−1 |
1-ATT |
0.7/0.1 |
6.5/8.7 |
−21.1/−6.8 |
15.7/43.3 |
−36.8/−50.1 |
|
n |
log Ks |
ΔrH°/kJ mol−1 |
TΔrS°/kJ mol−1 |
ΔrG°/kJ mol−1 |
1-rA-dT |
0.1 |
7.8 |
−6.3 |
38.4 |
−44.7 |
6-ATT b |
0.7 |
7.6 |
- c |
- c |
- c |
6-dA-rU b |
0.8 |
8.0 |
- c |
- c |
- c |
a Processing of titration data using Scatchard [66][67] equation gave values of ratio n [bound compound]/[polynucleotide]; correlation coefficients were >0.99 for most of calculated Ks; accuracy of n ± 20%, consequently log Ks values vary in the same order of magnitude; b I/I0 for 6-ATT and 6-poly dA−poly rU = 0.2 and 0.3; I0—starting fluorescence intensity of compound; I—fluorescence intensity of compound/polynucleotide complex calculated by Scatchard [66][67] equation; c Binding affinities were obtained by fluorescence spectroscopy. d All measurements were done at pH = 7.0 (buffer sodium cacodylate, I = 0.05 mol dm−3).
Binding affinities of
5 and
6 could not be calculated by ITC titrations due to the aggregation of these compounds in the ITC method concentration range that interfered with obtaining reliable results. For
6, binding affinities were assessed by fluorescence spectroscopy, which enabled measurements in lower sample concentrations. The fluorescence changes of
5 in titration with ATT triplex were too small for the accurate calculation of the binding constant. ITC titration experiments resulted in mostly negative peaks, indicating that the binding processes were exothermic (
Figure 4). The resulting data for the
1-poly rA−poly dT complex was fitted to a single-site binding model, while data for
1-ATT was fitted to model two sets of sites by using a nonlinear least square method (
Table 2). The analysis of ITC experiments of compound
1 with poly rA−poly dT and ATT triplex demonstrated high and similar binding affinities (log
Ks,
Table 2), which is consistent with the results from competitive dialysis assay. Compound
1 demonstrated two types of binding in titration with ATT triplex (
Figure 4). The first binding event was characterized by a higher binding constant than the second type of binding. The first binding event is an entropically guided process probably accompanied by the release of bound water molecules from the polynucleotide groove to the bulk
[64]. The second type of binding, characterized by a higher ratio N, is an enthalpy-driven process accompanied by an increase in the number of hydrogen bonds, aromatic stacking, electrostatic interactions, and van der Waals interactions, followed by a large favorable entropy contribution. The interaction of
1 with poly rA−poly dT was, similar to its complex with ATT, characterized by positive (favorable) binding entropy and weak negative enthalpy (
Table 2), indicating an entropically driven process. The groove binding is usually entropically favorable and slightly endothermic, resulting from the release of relatively highly ordered water molecules surrounding the apolar surfaces to the bulk. The enthalpy contribution to the free energy is associated with the overall increase of bonding (hydrogen bonds, ionic, electrostatic and van der Waals interactions, and polarization of the interacting groups)
[68]. An analysis of the fluorescence data of
6 with poly dA−poly rU and ATT polynucleotides gave high binding constants and rather high ratios, N, for both complexes. Unlike
6,
5 induced small emission changes upon binding to ATT triplex, thus disabling the calculation of the stability constant.
3.3. Thermal Melting Experiments and RNase H Assay
Thermal denaturation is an additional method for monitoring binding to nucleic acids
[69]. Stabilization of nucleic acid structure induced by small molecules causes an increase in the melting temperature of that structure. In a typical experiment, a ligand is monitored against one nucleic acid structure at conditions close to the equimolar ligand/nucleic acid ratio. The melting of mixtures, a relatively straightforward extension of the typical thermal melting experiment design, enables an evaluation of the stabilization effect of the ligand against a number of different nucleic acid structures. If there is an excess of nucleic acid over the compound where the binding sites are not fully saturated, a preference toward sequence or structure can be established
[63]. In this experiment, ligand selectivity was studied with a mixture of four different polynucleotides, DNA (poly dA–poly dT), RNA (poly rA–poly rU), and two DNA:RNA hybrids (poly rA–poly dT and poly dA–poly rU) (
Figure 5,
Table 3).
Figure 5. Melting profiles of polynucleotide mixtures (first derivative profile of absorbance versus temperature). Each panel shows the melting of a mixture of 4 polynucleotides: a DNA:RNA hybrid [poly dA−poly rU; peak 1], RNA [poly rA−poly rU; peak 2], an RNA:DNA hybrid [poly rA−poly dT; peak 3] and DNA [poly dA-poly dT; peak 4] as the solid black line (−). The concentration of each polynucleotide structure was 20 μM (bp); total polynucleotide concentration is 80 μM (bp). The dashed line (- - -) in each panel shows the effect of the addition of ligand. (A) 6 (B) 5 (C) 1 all at 2 μM (50 mM sodium cacodylate buffer, 50 mM NaCl, and 1 mM EDTA).
Table 3. The ΔTm a values (°C) of studied mixture of four polynucleotides (poly rA−poly dT, poly rA−poly rU, poly dA−poly rU, and poly dA-poly dT) b upon addition of ratio r = 0.025 c of 1, 5, and 6 d.
|
ΔTm a |
Polynucleotide |
1 |
5 |
6 |
poly dA−poly rU |
5.6 |
4 |
5.5 |
poly rA−poly rU |
2.2 |
1 |
0 |
poly rA−poly dT |
1.7 |
1.7 |
1.5 |
poly dA-poly dT |
19.1 |
2.1 |
0 |
a ΔTm = Tm (complex polynucleotide-dye) − Tm (polynucleotide); error in ΔTm: ±0.5 °C; b Tm values for mixture of polynucleotides without compound, poly dA−poly rU = 47 °C; poly rA−poly rU = 57.4 °C; poly rA−poly dT = 65.3 °C; poly dA-poly dT = 70.6 °C.; c r = [compound]/[polynucleotide]. d All measurements were done at pH 7.0 (sodium cacodylate buffer with NaCl, I = 0.1 mol dm−3 + 1 mM EDTA).
The compounds
1 and
5 demonstrated the stabilization effect of all studied ds-polynucleotides (
Table 3,
Figure 5). In addition, these two compounds demonstrated a better stabilization effect of poly dA−poly rU than of poly rA−poly dT. Interestingly, among ds-nucleic structures,
6 almost exclusively stabilized poly dA−poly rU. It has been demonstrated that the stability of hybrid duplexes, composed of homopurine and homopyrimidine strands, depends on several factors, such as the percentage of deoxyribo(pyrimidine), i.e., d(Py) content in each of the strands, the oligomeric length and the percentage of (A)
n:(T or U)
n content
[70]. Thus, for example, the hybrid duplex containing the DNA purine strand and the RNA pyrimidine strand d(Pu):r(Py), such as poly dA−poly rU, demonstrates much less thermal stability compared to the r(Pu):d(Py) composition, such as poly rA−poly dT. This could be a reason for the higher stabilization effect of dArU, in comparison to rAdT, induced by
1,
5, and
6. Among ds-polynucleotides,
1 demonstrated the most significant stabilization effect with poly dA−poly rU and especially poly dA−poly dT (
Table 3). It should be emphasized that
1 possesses a higher number of net positive charges than
5 and
6 (
1, +2;
5, +1;
6, +1). However, only
6 demonstrated selective stabilization of the hybrid duplex, poly dA−poly rU, in a mixture of double-stranded polynucleotides. As all three ligands stabilized poly rA−poly dT and poly dA−poly rU, we performed a spectrophotometric RNase H assay to evaluate the capability of
1,
5, and
6 to inhibit RNase H
[54]. The cleaving of the RNA strand from a DNA:RNA hybrid duplex was followed by an increase in A
260 (
Figure 6).
Figure 6. Inhibition of RNase H digestion of poly rA−poly dT by 1, 5, and 6; concentration of nucleic acid hybrid structure was 10 μM (bp); 1, 5, and 6, all at 3 μM.
The best inhibition activity of the RNase H digestion of poly rA−poly dT demonstrated benzothiazole 1. The data from a thermal melting of polynucleotide mixtures show a good correlation with the results of the RNase H assay. The RNase H assay was utilized here as an additional method to the thermal melting of polynucleotide mixtures. It provides information on which ligands can be biologically relevant as RNase H inhibitors and deserves detailed investigation regarding that. The melting profile of free ATT in sodium cacodylate buffer (50 mM sodium cacodylate buffer, 50 mM NaCl, and 1 mM EDTA) demonstrated biphasic transition (Figure 7, Table 4).
Figure 7. (A): Melting curve of ATT triplex upon addition of ratio, r ([compound/[polynucleotide]) = 0.1 of 1, 5, and 6 at pH = 7.0 (sodium cacodylate buffer with NaCl, I = 0.1 mol dm−3 + 1 mM EDTA; orange arrows—first transition, violet arrows—second transition); (B): First derivative of absorbance at 260 nm in dependence of temperature.
Table 4. The ΔTm a values (°C) of ATT triplex b upon addition of ratio c r = [compound]/[polynucleotide] of 1, 5, and 6 d.
|
ΔTm a |
|
1 |
5 |
6 |
cr |
0.1 |
0.2 |
0.3 |
0.1 |
0.2 |
0.3 |
0.025 |
0.05 |
0.1 |
|
31.7/21.9 |
32.5/23.9 |
- |
27.3/0 |
30.1/0 |
32.8/0 |
36.7/0 |
42.0/0 |
43.7/0 |
a ΔTm = Tm (complex polynucleotide-dye) − Tm (polynucleotide); error in ΔTm: ±0.5 °C; b Tm values for biphasic thermal denaturation profile of ATT without compound, Tm1 = 22.6; Tm2 = 70.7; c r = [compound]/[polynucleotide]; d All measurements were done at pH 7.0 (sodium cacodylate buffer with NaCl, I = 0.1 mol dm−3 + 1 mM EDTA).
The first transition was at
Tm1 = 22.6 °C and corresponds to dissociation of the third strand (poly dT with Hoogsteen base pairs from the major groove). The second transition was at
Tm2 = 70.7 °C and corresponds to dissociation of Watson–Crick base pairs of double-stranded poly dA-poly dT
[71][72]. Albeit
1 stabilized both the ATT triplex and poly dA−poly dT duplex, the higher stabilization effect was demonstrated with the ATT triplex. Unlike
1,
5 and
6 increased
Tm1, while
Tm2 did not change. Particularly interesting was compound
6, which stabilized ATT significantly even at very low ratios,
r (
Figure 7). Data from this experiment for poly dA−poly dT (
Table 4) duplex agrees very well with those for the same polynucleotide obtained in the melting of mixtures experiment (
Table 3).
3.4. Circular Dichroism (CD) Experiments
Based on competition dialysis results, we further proceeded with the characterization of selected complexes by CD spectroscopy. Electronic circular dichroism (ECD) is highly sensitive toward conformational changes in the helical structure of DNA and RNA and their complexes with small molecules
[73][74]. In addition, an induced CD spectrum (ICD) that can arise from the interaction of achiral small molecules such as
1,
5, and
6 with nucleic acids, could be very informative of the binding modes. We monitored changes in CD spectra upon the interaction of
1,
5, and
6 with representatives of the B-helix family, ctDNA with a mixed base pair composition, and with poly dA−poly dT characterized by a much narrower and deeper minor groove in comparison to other common B-helices. As a model of A-helical structure, we used poly rA−poly rU (ds-RNA) characterized by a wide and shallow minor groove and deep and narrow major groove
[75][76]. Interactions were also studied with two double-stranded DNA:RNA hybrids, poly dA−poly rU, and poly rA−poly dT and the DNA triple helix, ATT. While NMR, Raman, and X-ray fiber diffraction studies suggest B-like conformation for poly rA-poly dT in high humidity conditions, it seems that the global conformation of poly dA-poly rU is considerably more affected by the ribopyrimidine strand, resulting in an A-type helix closer in conformation to the A-form of RNA
[77][78]. Further, NMR and IR data suggest that structural characteristics of triplexes resemble B-DNA much more than A-DNA
[79][80][81]. The addition of
1,
5, and
6 to polynucleotides mainly caused a decrease of CD intensity of DNA and RNA polynucleotides at their maximal values (ctDNA at 275 nm, AT-DNA, AU-RNA, and DNA:RNA hybrids at 260 nm) (
Figure 8); however, induced CD spectra of these compounds, which changed with increasing ratio,
r, were more informational regarding their modes of binding.
Figure 8. CD titrations of (a) poly rA−poly dT, (b) poly dA−poly dT, (c) ATT triplex, (d) poly dA−poly rU, (e) poly rA−poly rU, and (f) ctDNA (c = 3.0 × 10−5 mol dm−3) with 6 at molar ratios r = [compound]/[polynucleotide] = 0.3 (pH = 7.0, buffer sodium cacodylate, I = 0.05 mol dm−3 + 1 mM EDTA for all titrations except poly dA−poly rU (I = 0.2 mol dm−3 + 1 mM EDTA)).
At
r ≤ 0.1,
6 caused either negligible or weak negative ICD signals (around 320 nm) with all polynucleotides. However, at
r > 0.1, this compound differentiates between B- and A-type helices by the mode of binding (
Figure 8). Negative ICD signals support intercalation to A-type helices, poly dA−poly rU and poly rA−poly rU, whereas an appearance of a bisignate CD signal implied the formation of
6 dimers most probably inside the minor groove of poly rA−poly dT, poly dA−poly dT, and ATT triplex. Interactions of
6 were additionally investigated with ATT 26mer by fluorimetric and CD spectroscopy to estimate the influence of the ATT polymer length on the binding strength. Processing of titration data gave ratio
n and binding constant (log
Ks = 7.4,
n = 0.7) comparable to that obtained with the ATT polynucleotide (
Table 2). Regarding CD titration of
6 with ATT 26mer, a similar bisignate ICD signal, as in the CD titration with ATT polynucleotide, was noticed, suggesting that ATT polymer length did not influence the mode of binding
[82]. Unexpectedly,
6 did not bind in the form of dimers to ctDNA (B-helix), instead, changes (negative ICD signals,
Figure 8) were indicative for intercalation, which can probably be related to the composition of ctDNA containing 42% of GC basepairs beside AT base pairs. Unlike
6,
5 did not differentiate among polynucleotide conformations. Its addition caused a rise of bisignate ICD signals with all studied nucleic acid structures. Such an effect supports a formation of dimers (minor groove of ATT triplex and major groove of poly rA-poly rU) or larger aggregates, similar to those observed with poly dA-poly dT and ctDNA. On the other hand, bis-benzothiazolyl-pyridine
1, which is sterically more demanding compared to
5 and
6, provoked a strong positive ICD band (at 340 nm) at
r ≤ 0.1, implying binding within the minor groove of ctDNA, poly dA−poly dT, and ATT triplex (
Figure 8). At ratios higher than
r = 0.1, an excess of
6 molecules cannot accommodate inside the minor groove so well; instead,
6 forms aggregate along the polynucleotide backbones. Negative ICD signals (around 340 nm) at
r ≤ 0.1 point towards intercalative binding to poly rA−poly rU and both hybrids. An increase in ICD intensity with an increase of
r (
r > 0.1) suggests aggregation of
6 along the polynucleotide surfaces. Further, a clear isodichroic point (λ = 253 nm) observed for
1 and poly rA−poly dT strongly suggests one dominant interaction mode of this compound with the DNA chiral axis.
Due to the strong interaction of 1 with the ATT triplex, we decided to examine interactions of this triplex with its regioisomer 3 to see whether the position of benzothiazole-imidazolinyl chains on pyridine ring affected them. Interestingly, compound 3, unlike its regioisomer 1, induced a strong increase in the intensity of CD spectra of ATT triplex, poly dA-poly dT, and ctDNA (ctDNA at 275 nm, AT-DNA at 260 and 282 nm, and ATT at 282 nm) compared to the intrinsic CD bands of these polynucleotides, and in addition, strong positive ICD bands located around 350 nm (Figure 9, SI).
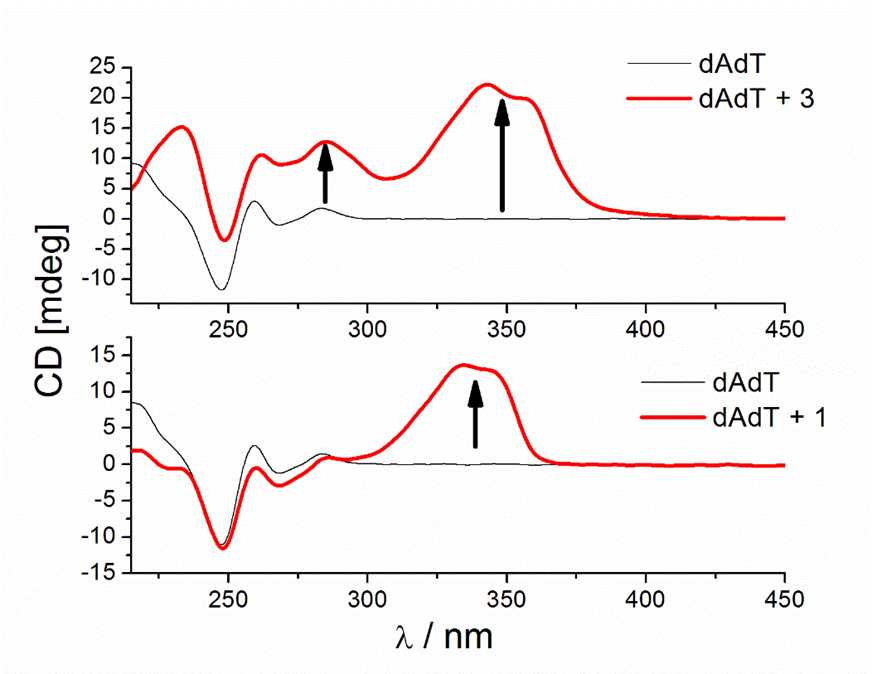
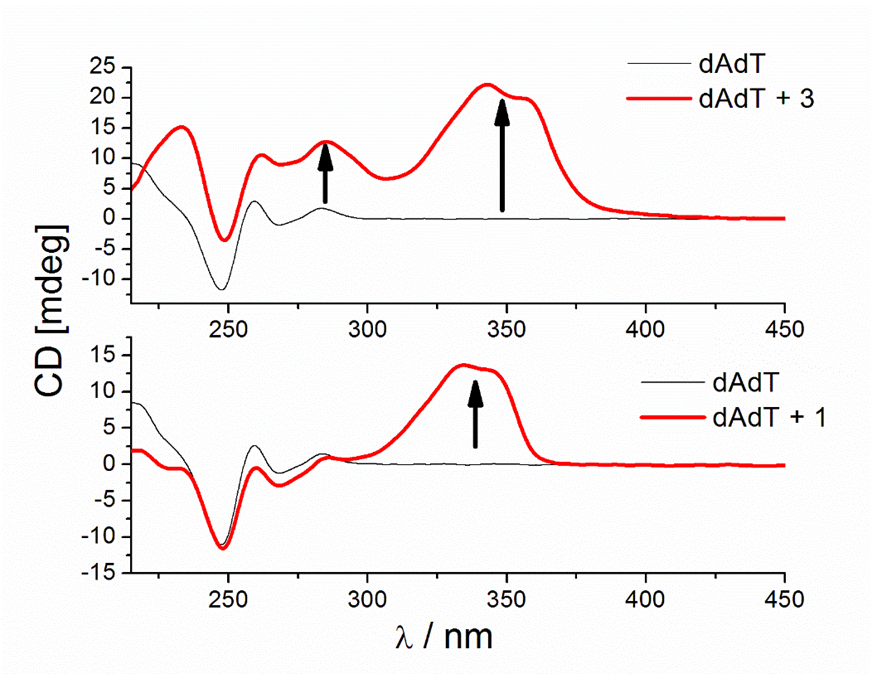
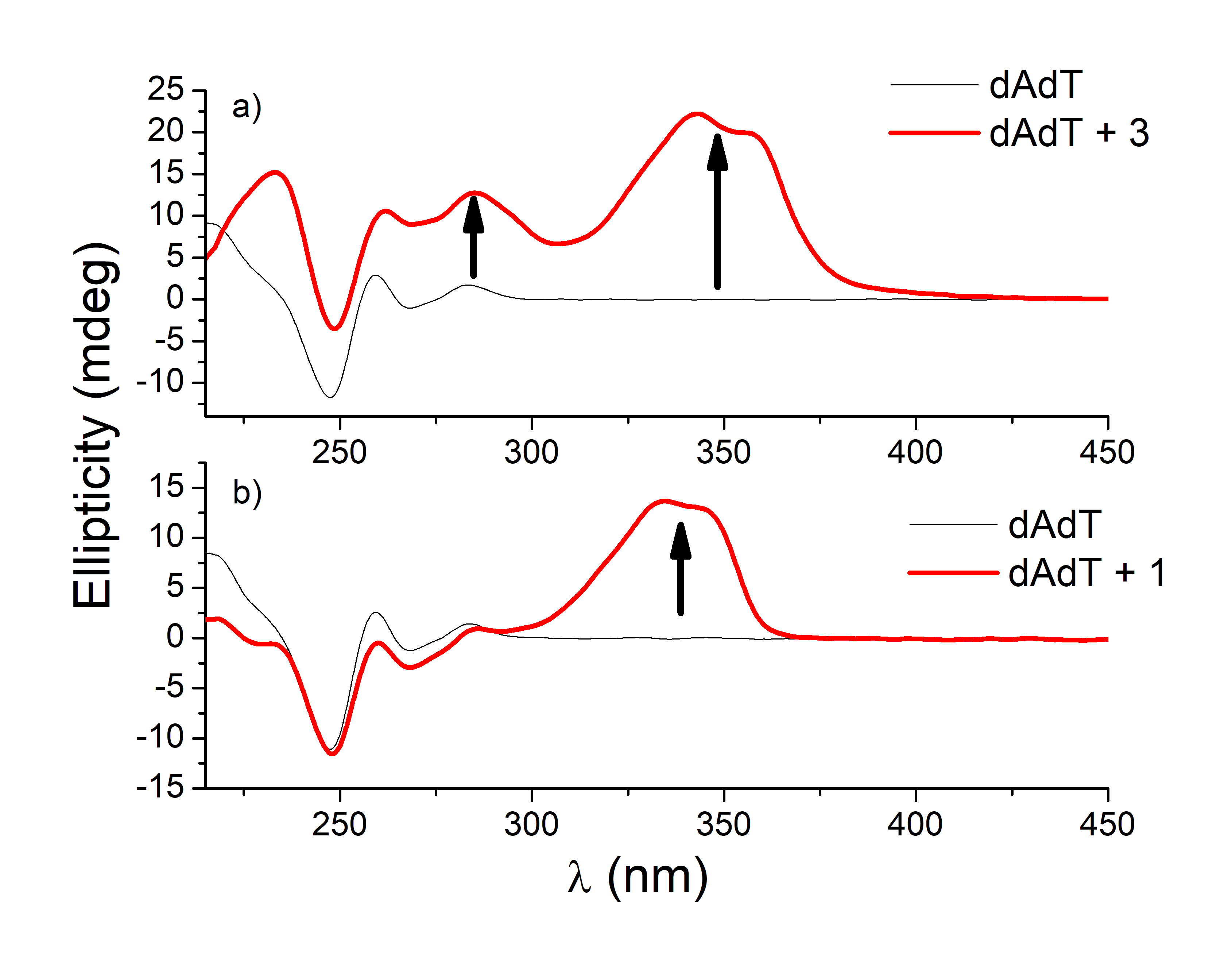
Figure 9. CD titrations of AT-DNA duplex (dAdT) (c = 3.0 × 10−5 mol dm−3) with 3 (a) and 1 (b) at molar ratios r = [compound]/[polynucleotide] = 0.4 and 0.1, respectively (pH = 7.0, buffer sodium cacodylate, I = 0.05 mol dm−3 + 1 mM EDTA).
Similar strong changes in CD spectra of polynucleotides were noticed with bis-polyaza pyridinophane derivatives that, similarly to compound 3, consist of two chains attached to pyridine as a central unit [66]. The reason that compound 3 induced condensation-like changes, while compound 1 did not, is probably the position of chains on the pyridine ring. Unlike 1, 3 and bis-polyaza pyridinophane derivatives have two chains attached at the same positions on the pyridine ring (2 and 6 positions).
3.2.5. Molecular Modeling
The binding of 6 dimers inside the minor groove of the ATT triplex was also examined by molecular modeling (Figure 10). The mode of binding of 6 with the ATT triple structure suggested by the spectroscopic methods was consistent with the results obtained by molecular modeling.
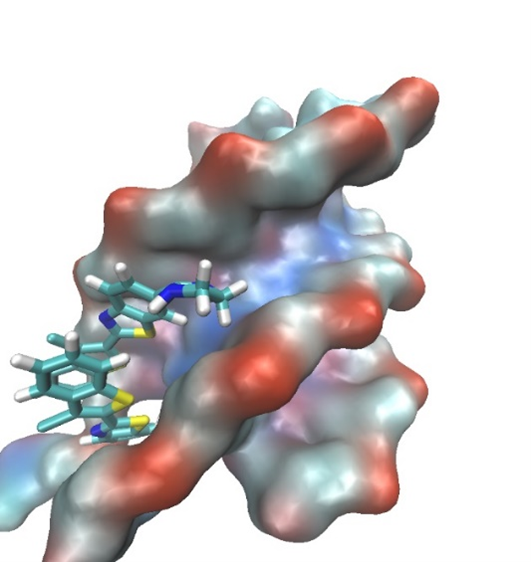
![Figure 10. A complex between ATT (DNA triplex) and dimer of 6 obtained after 200 ns of MD simulation in water. ATT is represented by its solvent accessible surface and ligand is given in stick representation. (complexes were built in PyMOL, wherein the initial position of ligands was de-termined from spectroscopic data. Parametrization was performed by ANTECHAMBER [67] and Leap, the modules available within AMBER16 suite of programs [68,69] using GAFF [70] for the ligands and (a) bsc1 [41] and (b) OL15 [69] for ATT. Neutralized and solvated complexes were minimized, equilibrated, and simulated for 200 ns using the programs sander and pmemd.](/media/item_content/202203/figure10a-6229cf3c08d89.tiff)
- Conclusions
The search for small molecules with selective binding to specific sites in DNA or RNA structures is still of intense interest. Such binding can block or interfere with important processes, e.g., transcription, recombination, and DNA repair.
Competition dialysis assay [27,71,72] allows the straightforward evaluation of sequence and structural selectivity of different DNA and RNA binding ligands. In this study it enabled the detection of three benzothiazole compounds, (1, 5, and 6), with preferential binding to DNA:RNA hybrids and ATT triplex in regard to regular (non-hybrid) DNA and RNA duplexes and single-stranded forms. Compound 6 preferentially stabilized dArU hybrid among other ds-polynucleotides. RNase H assay confirmed the results of thermal melting experiment (Table 3) and identified ligand 1 as a potential RNase H inhibitor for the digestion of poly rA-poly dT. While all three compounds demonstrated a strong stabilization effect of ATT triplex, only compound 6, in both thermal melting experiments (Tables 3 and 4), demonstrated selective binding to ATT triplex in regard to AT duplex. Such stabilization could be exploited to inhibit the gene expression involved in cancer, for interference with DNA replication or inducing transcriptional repression, site-specific mutations, and recombination [11].
To the best of our knowledge, among small molecules previously reported [73–77], 6 with the chlorobenzothiophene substituent has the largest stabilization effect on ATT triplex (DTm = 44 at r = 0.1). In addition, 5 (with bithiophene substituent) and, especially, 6 selective stabilization of triplex in the form of dimer inside the minor groove, has not been reported yet. The mode of binding of 6 (inside the ATT minor groove in the form of dimer) was confirmed by CD spectroscopy and molecular modeling. These results were made with long polynucleotides (≥500 base pairs) that can provide a large excess of binding sites along the DNA helical structure and ease the determination of binding modes. To see if the same selectivity of molecule 6 toward ATT base triplets exists in shorter sequences, we performed measurements with the 26-nucleotide-long ATT triplex, possessing the same conformation (B-DNA, Figure 10) as its longer form. The fluorescence and CD experiments performed with 26 mer revealed high affinity and the same ICD profile as with longer sequences, confirming that the pattern of recognition is present regardless of nucleotide chain length.
As the AT-rich repeated sequences are often found in the sites for DNA replication initiation in bacterial, archaeal, and eukaryotic replicons, it would be interesting to confirm identified selectivity with a more distinct AT-rich oligomer sequence, for example, the GATCTATTTATTT of replication origin in E. coli [78]. However, this will be investigated in another study, after careful selection of the oligomers. Compound 6 could differentiate between B- and A-type helices by the mode of binding. While an appearance of negative ICD signals supported intercalation to A-type helices, poly dA-poly rU, and poly rA-poly rU, bisignate CD signal implied the binding of 6 in the form of dimers inside the minor groove of poly rA-poly dT, poly dA-poly dT, and ATT triplex.
In contrast to its regioisomer 1, ligand 3 induced a strong increase in CD intensity of AT-rich sequences (ctDNA at 275 nm, AT-DNA at 260 and 282 nm, and ATT at 282 nm) and strong positive ICD bands around 350 nm (SI). Similar condensation-like changes of the nucleic acid structure could be utilized in pharmaceutics for DNA delivery by viral or non-viral vectors in gene therapy [79–81]. Furthermore, DNA condensation can be applied in the construction of biosensors based on the liquid-crystalline properties of condensed DNA [82]. This result, as well as the identification of ligand 1 as a potential RNase H inhibitor, will be investigated in more detail and will be published elsewhere.