Optic flow was first introduced by Gibson as part of their ecological approach to perception and action. While this theoretical approach (as a whole) has been demonstrated to be particularly suitable for the study of goal-directed displacements in humans, its usefulness in carrying out entomological field studies remains to be established.
1. Perceptual Information Used in Flight Control in Insects
Over the last eighty years, it has often been shown that flying insects rely on optic flow to perform various locomotion tasks
[1][2][3][4][5]. Optic flow can be defined as a vector field of the apparent motion of objects, surfaces, and edges in a visual scene caused by the relative motion between an agent and the scene while being independent of the scene’s texture (see
Figure 1 and
[6][7][8]). Insects seem to be masters of spacial navigation due to their ability to extract perceptual information from optic flow in order to finely control their everyday locomotion tasks: terrain-following, centering, wall-following, speed adjustment, and landing (see review articles
[3][4][5][9]).
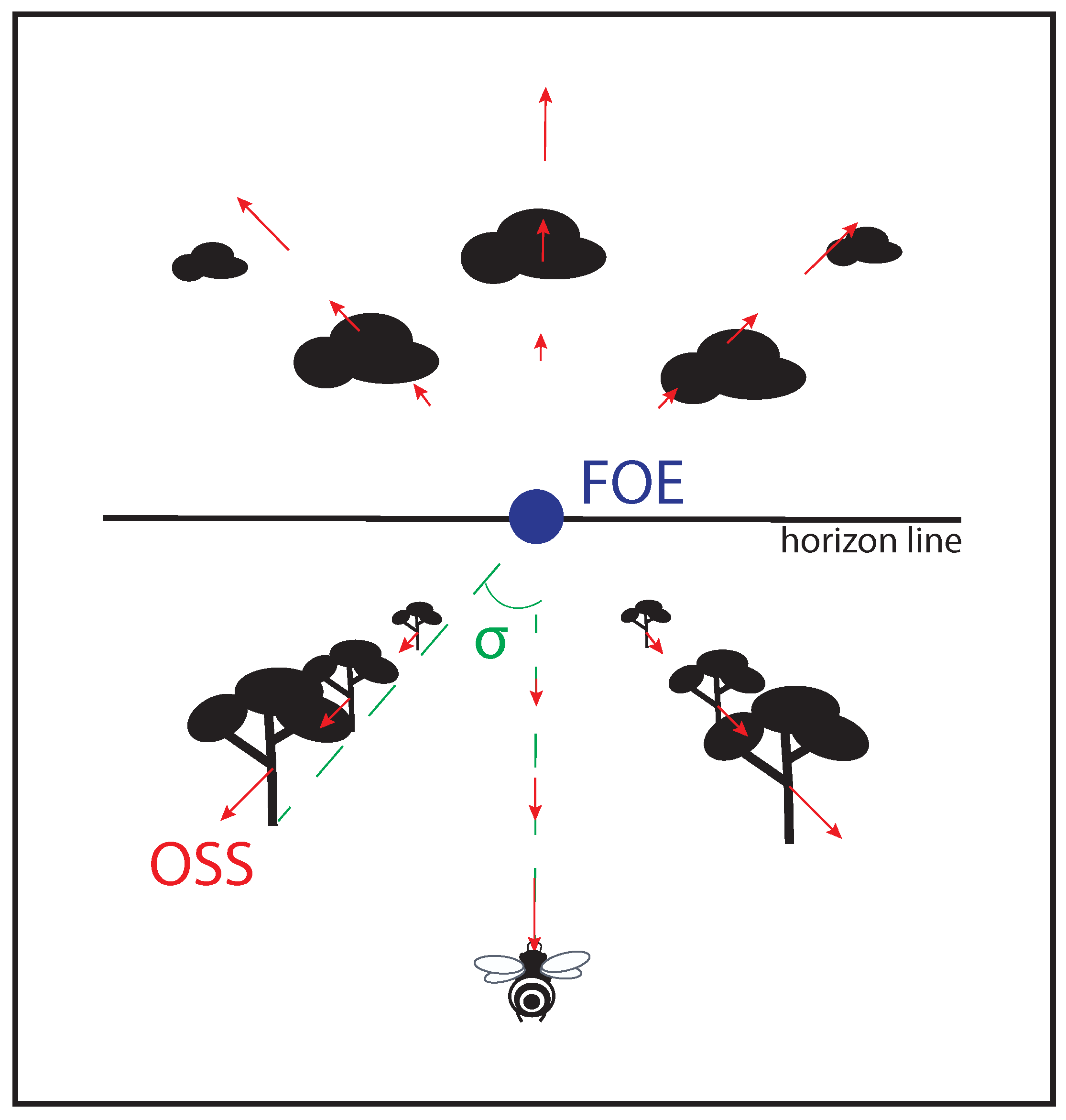
Figure 1. Non exhaustive optic flow variables perceived by a flying agent. Objects sliding on the optical sensor of an agent bear an optical velocity (OV) depending on the distance agent-object, and on the agent’s speed; focus of expansion (FOE) at the center of the visual field is the only point providing no OV; splay angle (
σ
) is the angle formed by the direction vector of the agent and one of the lines parallel to the movement joining the horizon.
-
Terrain-following task: The ventral part of the optic flow is useful to follow the ground
[3][10][11][12][13][14][15][16][17]. Srinivasan et al.
[11] proposed “the image velocity of the ground is held approximately constant” to achieve a terrain-following behavior. Portelli et al.
[13] proposed: “(1) honeybees reacted to a ventral optic flow perturbation by gradually restoring their ventral optic flow to the value they had previously perceived, (2) honeybees restored their ventral optic flow mainly by adjusting their flight height while keeping their airspeed relatively constant”. However, Straw et al.
[18] “found that flies do not regulate altitude by maintaining a fixed value of optic flow beneath them”, but “
Drosophila flies establish an altitude set point on the basis of nearby horizontal edges and tend to fly at the same height as such features”. A similar conclusion was drawn by David
[10] about altitude regulation: “flies did not adjust the angular velocity of image movement that they held constant” for speed control purposes.
-
Centering and wall-following tasks: The lateral/frontal parts of the optic flow are useful to center in a narrow corridor by balancing the lateral parts of the optic flow (in honeybees
[3][19][20], in bumblebees
[15][21], in flies
[10][22], in hawkmoths
[23]), or to follow a wall along a wide corridor by restoring the optic flow pattern from one side (in honeybees
[19][20][24], in bumblebees
[25]). Kirchner and Srinivasan
[24] suggested “bees maintained equidistance by balancing the apparent angular velocities of the two walls, or, equivalently, the velocities of the retinal images in the two eyes”. Dyhr et al.
[25] found a similar conclusion in bumblebees: “the centering response relies on a direct comparison of the optic flow from each eye providing a more accurate measure of the perceived differences”. Lecoeur et al.
[15] found that “lateral position is controlled by balancing the maximum optic flow in the frontal visual field”. Stöckl et al.
[23] found that “hawkmoths use a similar strategy for lateral position control to bees and flies in balancing the magnitude of translational optic flow perceived in both eyes”. Serres et al.
[20] found that “bee follows the right or left wall by regulating whichever lateral optic flow (right or left) is greater”.
-
Speed adjustment task: The bilateral (or bi-vertical) part of the optic flow is useful to adjust flight speed
[3][21][26][27] (or
[28]). Srinivasan et al.
[26] showed that honeybees decrease their flight speed in a narrowing tunnel, and increase it as the tunnel widens. The authors of this study suggested that the visuomotor strategy consists of “holding constant the average image velocity as seen by the two eyes” without specifying any part of the visual field. A similar conclusion was drawn in flies
[10][29]. Baird et al.
[27] confirmed these results by manipulating the bilateral part of the optic flow and concluded that “honeybees regulate their flight speed by keeping the velocity of the image of the environment in their eye constant” by taking into account both the lateral and the ventral part of the optic flow. Portelli et al.
[28] found in honeybees “that the ground speed decreased so as to maintain the larger of the two optic flow sums (”left plus right“ optic flows or ”ventral plus dorsal“ optic flows) constant according to whether the minimum cross-section was in the horizontal or vertical plane”.
-
Landing task: The ventral part of the optic flow can also be regulated by honeybees to land
[11][26]. Srinivasan et al.
[11] concluded that honeybees “tend to hold the angular velocity of the image of the surface constant as they approach it” in order to adjust their height above a flat surface. Srinivasan et al.
[11] also concluded that “the bee decelerates continuously and in such a way as to keep the projected time to touchdown constant as the surface is approached”. This “projected time” stringently means a time-to-contact (TTC). This procedure ensures the agent’s speed decreases proportionally with the distance to the ground, reaching a value near zero at touchdown
[3][5][9].
As stated previously, most of the studies cited in this first section highlight the central role played by optic flow in the control of navigation tasks in insects. A close inspection of these studies demonstrates significant disparities between the terms used when referring to the same concepts or entities (see
Table 1). It also reveals, in some cases, confusions between the perceptual support used by insects in the control process and the property of the environment to which it gives access. We would like to argue that these disparities and confusions reveal a need for some theoretical clarifications. To be fully useful by the entomologist community, the optic flow concept must be used in the same way as in its original theoretical framework, i.e., the ecological approach of perception action proposed by Gibson in the last century
[6]. Gibson’s framework has already proved to be relevant in addressing issues related to the control of human displacement
[30][31] and by offering the robotics community a framework for carrying out new generations of studies
[32][33]. We would like to argue in this contribution that the ecological approach to perception and action, taken as a whole, provides powerful theoretical and methodological tools allowing the entomologist community to: (i) take a critical look at the research carried out to date, (ii) develop more rigorous and innovative experimental protocols, and (iii) define new scientific issues pushing the boundaries of the current research field.
Table 1. Variability of denominations used in the entomologist community to describe perceptual information.
Task |
Denomination of the Perceptual Information in Entomology |
Species |
Centering and wall following |
(lateral) “image angular velocity” in [34] “horizontal optic flow cues” in [34] “speed of retinal image motion” in [26] “apparent angular speed” in [26] “lateral optic flow” in [20] “the magnitude of translational optic flow perceived in both eyes” in [23] “translational optic flow cues” in [35] |
Apis mellifera, Bombus terrestris, Megalopta genalis, Macroglossum stellatarum |
Speed adjustment |
(lateral) “image angular velocity” in [11][27] “optic flow cues in the lateral visual field” in [27] “velocity of the perceived image motion” in [27] “rate of optic flow” in [21][27] “image motion signal” in [27] “optic flow cues” in [27] “apparent velocity of the surrounding environment” in [26] “apparent movement of the surrounding patterns relative to themselves” in [10] “retinal slip speed” in [29] |
Apis mellifera, Bombus terrestris, Drosophila hydei, Drosophila melanogaster |
Terrain following |
“ventral optic flow” in [13] “apparent (ventral) speed of image” in [26] “image angular velocity” in [27] “optic flow cues in the ventral region of the visual speed” in [27] “rate of optic flow” in [27] “perceived image velocity of motion of the image” in [27] |
Apis mellifera |
Landing on vertical surface |
“tau: apparent rate of expansion of the image” in [11] “magnitude of optic flow” in [36] “speed of image motion on the retina” in [36] |
Apis mellifera |
Landing on horizontal surface |
“angular velocity of the image” in [11][26] |
Apis mellifera |
Heading |
“apparent movement” in [1] “retinal image displacement” in [1] |
Aëdes aegipty |
2. How the Ecological Approach Allows a Better Understanding of the Processes Underlying Trajectory Control in Insects
2.1. The Ecological Framework as a Transversal Framework
One of the cornerstones of the ecological approach proposed by Gibson lies in the fact that it applies indifferently, whether we are interested in the behavior of humans, insects, or robots. It is this idea that appears in the background when Gibson refers to the concept of agents and considers that the perceptual information used to control a given displacement is available in the perceptual flows in which agents are immersed, not in the head of an agent after a cognitive post treatment. If we push this reasoning to the extreme, despite having different sensory modalities, humans or insects could detect the same high order variable(s) in the perceptual flows when they perform similar tasks.
2.2. New Reading of the Data from the Literature
While the literature contains numerous results which demonstrate the major role played by optic flow in the control of displacement in insects, the conceptual framework provided by Gibson (see
Section 2) allows a more detailed analysis of the perceptual-motor processes implemented. As an example, when reviewing the different tasks mentioned in
Section 1, several clarifications can be made.
-
Terrain-following tasks: The studies mentioned
[3][10][11][12][13][14][15][16][17] indicate that honeybees rely on the value of optic flow velocity (sometimes called global optic flow rate), a low order variable, to maintain their height. The key point in this particular task is that the high order variable is the OVRC (Optical Velocity Rate of Change), and not the value of the OV (Optical Velocity) per se. Indeed, as OV is a ratio of speed over distance to the ground, further combinations of height and speed can provide the same OV value. In the case of forward displacement speed being constant, any variation in the OVRC “tells” the insect that the height is changing and requires a change in altitude. A close coupling between a high order variable and an action parameter allows the terrain-following task to be performed.
-
Centering task: The centering behavior observed in many insect species
[3][10][15][19][20][21][22][26] arises, in all probability, from the detection of a high order variable: motion parallax. Motion parallax corresponds to the OV gradient following a displacement of the agent in the environment
[37]. This gradient makes it possible to locate the objects of the environment in relation to each other. When the two side walls have the same OV, they are equidistant from the observation point, i.e., the agent is moving along the center of the corridor. Equalizing the OV of the two walls guarantees the production of a centered displacement.
-
Speed adjustment task: The studies reviewed in
Section 1 seem to indicate that OVRC could be used as part of a safety principle. When a flight tunnel narrows or widens for a given displacement of the agent, the OV increases or decreases, respectively. CanOcelling any change in OV despite changes in tunnel section gives rises to a safe behavior, i.e., a decrease in forward displacement speed when the tunnel narrows, and an increase in displacement speed when the tunnel widens.
-
Landing task: Regardless of the fact that several high order variables can be used to control landing tasks, the study by Srinivasan
[11] is interesting because it allows us to distinguish, as part of the control of a landing task, between a high order variable (tau: Τ), the PAES (Property of the Agent-Environment System) it specifies (first order TTC) and how the high order variable can be used as part of a control strategy. Within the framework of the strategy described, maintaining the value constant is a sufficient condition for zeroing velocity displacement as the surface is approached.
τ
3. Conclusions and Perspectives
Herein is to promote the conceptual framework provided by Gibson
[38] to address issues related to the perceptual control of goal-directed displacements in insects. Herein has shown how the use of this framework could allow the entomologist community to have a more precise idea of the different perceptual degrees of freedom available, potentially useful for insects, in perceptual visual flows. The difference in the status of these perceptual degrees of freedom (low order vs. high order variables) would be worth considering in future studies. The ecological approach of perception and action also provides a very convenient methodological framework with a clear experimental agenda which would help to guide the researcher’s approach. Last but not the least, this approach would allow a renewal of questions and guide future research towards new scientific challenges.
This entry is adapted from the peer-reviewed paper 10.3390/insects12121075