Several additional treatments that could potentially supplement existing wastewater treatment plants (WWTPs) to eliminate pollutants include a range of physicochemical and biological methods. The use of enzymes, specifically, oxidoreductases, are increasingly being studied for their ability to degrade different classes of organic compounds. These enzymes have been immobilized on different supports to promote their adoption as a cost-effective and recyclable remediation approach. Unfortunately, some of these techniques have shown a negative effect on the enzyme, including denaturation and loss of catalytic activity.
1. Enzyme Immobilization
Enzyme immobilization is defined as the attachment of an enzyme to an inert insoluble support material that will lead to the reduction or total loss of mobility of the immobilized enzyme. It has many advantages, including stability, reusability, the ability to recover enzymes, and enhanced tolerance of pH and temperature
[1][2][3]. Gholami-Borujeni et al. reported that immobilized horseradish peroxidases (HRPs) on calcium alginate cell beads were more stable in regards to pH and temperature than free HRPs when degrading acid orange 7 dye
[4]. On the other hand, enzyme immobilization can cause issues, such as a decrease in enzymatic performance, because of confirmational changes in the enzyme, steric hindrance, and mass transfer limitation
[5][6][7]. For these reasons and more, there is a need to develop new methods and new materials that overcome the shortcomings of different immobilization methods. There are two primary immobilization techniques. One technique is to physically attach the enzyme to the support materials, and the second technique is chemical binding. Under these two techniques, there are four different methods: entrapment, adsorption, covalent attachment, and cross-linking.
Figure 1 shows these different methods for enzyme immobilization.
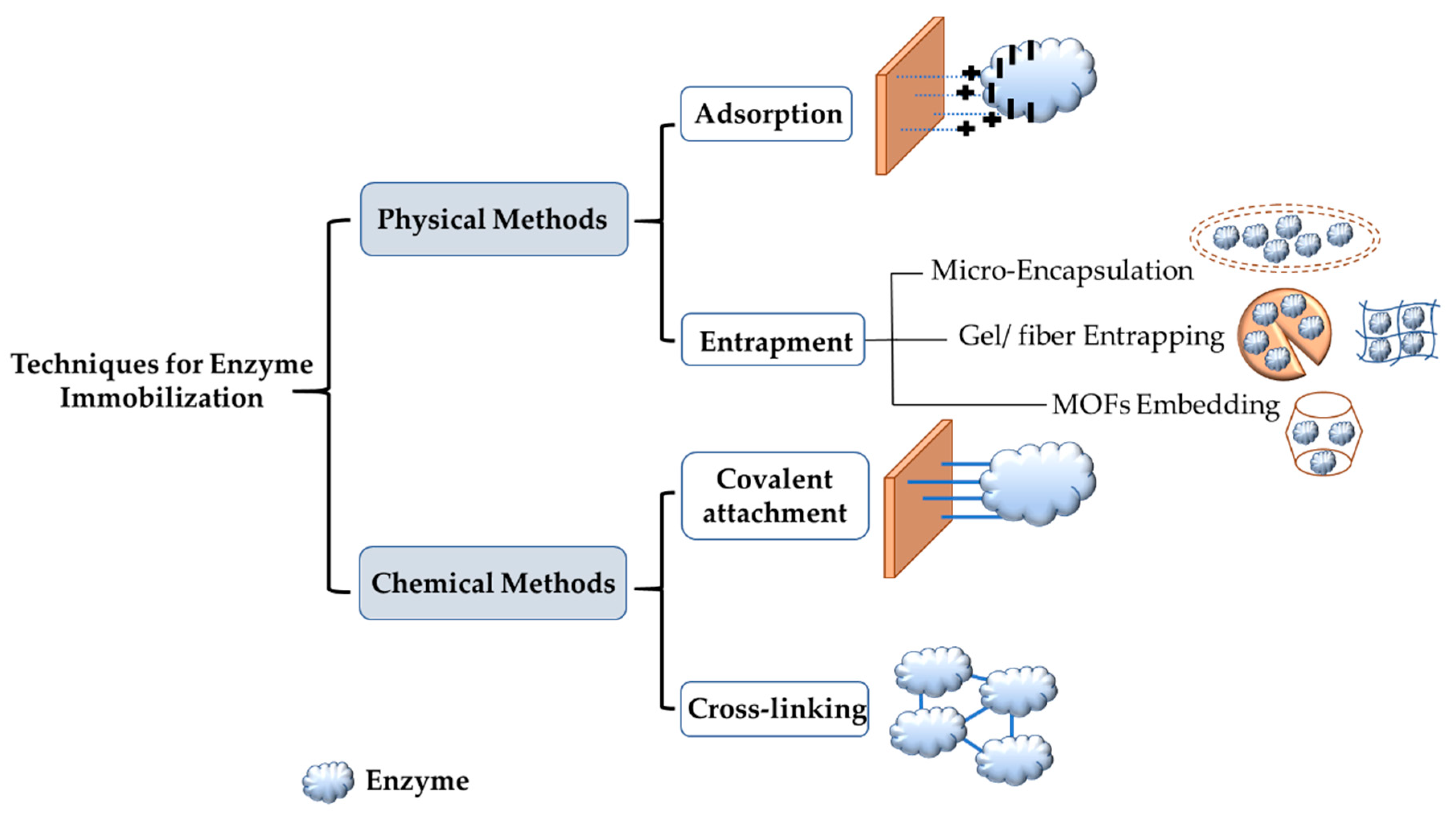
Figure 1. Commonly used approaches for enzyme immobilization.
Adsorption and entrapment are physical immobilization methods. These two methods depend on weak interactions, such as van der Waals forces and ionic binding between the support and the enzyme. Physical immobilization can help preserve enzyme activity since the native enzyme structure is not affected
[8]. In adsorption, the enzyme is physically bonded to the support surface via hydrogen bonds, van der Waals forces and ionic binding. This method is reversible and is the simplest one used for immobilization. It is also affordable, easy to prepare, and the most commonly used in industrial applications for scaling-up
[9]. Lee et al. demonstrated the immobilization of lipase enzyme on sol–gel dried silica using carbon nanotubes to protect the enzyme. The results revealed that immobilized lipase had better activity and a longer lifetime
[10]. The adsorption method can use inorganic and organic support materials. Inorganic support materials include silica. The type of silica used on a large scale is mesoporous silica SBA-15 with hexagonal arrays of pores and a pore size from 5 to 30 nanometers in diameter
[11][12]. Silica gels, which possess good thermal stability and mechanical strength, are also used as carriers. The particle size in silica gel is usually 70 to 150 μm
[13]. For organic carriers, chitosan, calcium alginate, cellulose, and agarose gel are used
[14][15]. For the adsorption of the enzyme on the support material to be completed successfully, specific functional groups should exist on both the enzyme and the carrier. If these groups do not exist, chemical modification can be performed to ensure successful immobilization. Chemical modification is performed using a modifying agent with at least two reactive groups. One of these groups is needed to attach the modifying group chemically to the support, and the other group is to physically interact with the enzyme for immobilization. One of the most commonly used modifying agents is glutaraldehyde, which has two aldehyde groups
[16][17][18]. Another physical enzyme immobilization technique is entrapment. This method, although physical, is not reversible. The enzyme is confined in a porous matrix support, thus allowing broad products to pass through while the enzyme cannot. Types of entrapment are metal-organic frameworks (MOFs), gel/fiber entrapment and microencapsulation. Entrapment leads to a significant increase in both thermal and storage stability due to the shielding and protection of the enzyme from denaturation under harsh conditions
[2][9]. Cui et al. showed that CPO immobilized on Fe
3O
3 magnetic nanoparticles using the entrapment method was successful in degrading more than 90% of aniline blue dye
[19].
Covalent attachment and cross-linking are chemical immobilization techniques. These techniques use a covalent bond to attach the enzyme and the support material using agents such as glutaraldehyde. This can lead to conformational change in the enzyme and blocking of the active site, thus affecting the activity of the enzyme. However, this is compensated for by strong chemical bonds and rigidity. The covalent attachment method can be defined as the formation of a covalent bond between the functional groups of the enzyme (including amino, carboxylic, and hydroxyl groups) and the support material used
[20]. Bilal et al.
[21] used covalent attachment to immobilize HRP on a calcium alginate support. The results showed that immobilized HRP had greater enzymatic efficiency and stability than free HRP. One of the reasons that this technique leads other immobilization techniques is that covalent attachment can stop enzyme leaching, thus preserving the enzyme. Another chemical immobilization method is cross-linking, which does not use support materials or matrix; instead, it develops intermolecular cross-linkage between the enzymes used by agents such as glutaraldehyde and diazonium salt
[20]. Sun et al. immobilized HRP on nanocomposites by cross-linking using diethylene glycol diglycidyl ether. Immobilized HRP showed improved durability, increased activity, reusability, and it withstood microbial attack
[22]. This technique has drawbacks that include enzyme denaturation in the immobilization process, which causes a loss of catalytic activity of the enzyme. Additionally, this technique has high operational costs and presents difficulties in controlling the reaction
[20].
2. Major Challenges and Recent Progress with Enzyme-Based Approaches
2.1. Stability
It is well recognized that the main challenge in using free enzymes at both laboratory and industrial scales is their low stability during storage or under harsh conditions, which include high and low pH, high temperature, organic solvents, ionic liquids and oxidizing agents such as H
2O
2 [23][24]. Nevertheless, enzyme immobilization as well as enzyme engineering and evolution are becoming powerful tools for the improvement of enzyme stability in different environments
[25][26][27].
2.1.1. Stability of Immobilized Enzymes
Various research groups have been attracted to immobilization processes as a means to overcome the low stability of free enzymes
[28][29]. Regarding pH, in most cases, immobilization has resulted in the unaltered activity of the immobilized enzyme at different pH values, a shift in the optimum pH, or a broader profile with little enhancement
[30][31][32][33]. Nevertheless, stability in other conditions could be greatly improved. Rong et al. synthesized a multiarmed magnetic graphene oxide (GO) composite (GO@Fe
3O
4@6arm-PEG-NH
2) as a carrier to immobilize HRP. The study investigated the storage stability of immobilized HRP. During cold storage at 4 °C, the immobilized HRP preserved 85.5% of its initial activity after 30 days and 72.5% after 60 days. On the other hand, free HRP sustained 42.3% of its activity after 30 days, and it was almost inactive (10.2%) after 60 days. Moreover, immobilization resulted in a substantial improvement in the thermostability of the enzyme
[30].
2.1.2. Enzyme Engineering and Evolution
With the development of recombinant DNA technology and enzyme engineering, generation of customized and evolved enzymes are now possible. In enzyme engineering, key mutations are introduced that improve the enzymes’ catalytic and biophysical characteristics. Such changes might lead to enhanced stability towards extreme temperature and pH, high substrate concentrations, and tolerating different organic solvents
[34]. Enzyme engineering strategies can be divided into two broad categories: directed evolution and rational design. In directed evolution, two main approaches are followed, one way by recombining related sequences randomly such as gene shuffling, the other way is by introducing random mutations in an enzyme such as error prone PCR. The advantages of such approaches are that they do not require structural information, and variations at unexpected positions can be introduced, which can be far from the active site.
[35].With all the advantages of directed evolution, it is accompanied with drawbacks. The changes that are produced by such approach are usually small and multiple rounds of evolution are required, which will result in a significant number of variants being screened and tested. This technique appears to be time consuming and requires reliable and high-throughput assays
[35].
2.2. Recyclability and Reusability
2.2.1. Immobilization on Membranes
Enzymes immobilized on synthetic or commercially available membrane supports have gained much attention in recent years. Some of the attractive properties of membrane support are a large surface area, which facilitates the attachment of enzymes, and pore size, good porosity, and structure. All these factors help the reaction mixture (contaminated wastewater) access the active sites of the enzymes
[36][37]. Moreover, membrane shape and geometrical configuration can be adjusted to their required purpose
[38]. More critically, membranes allow for the reusability of immobilized enzymes for multiple cycles. Handayani et al. immobilized lipase enzyme on a membrane to improve its reusability. They synthesized polyethersulfone (PES) and NH
2-polyethersulfone (PES–NH
2) for enzyme immobilization. Membranes with pore sizes ranging from 10 to 600 nm were fabricated based on polyethersulfone (PES) and NH
2-polyethersulfone (PES–NH
2) polymers to be used in a bioreactor to enhance the performance of the immobilized lipase enzyme. The activity of the immobilized enzyme was not significantly affected by the immobilization process. The reusability of this system was tested using a hydrolysis reaction between p-nitrophenyl acetate and methanol. The reusability test was then repeated four times. The results showed that the activity of the lipase enzyme immobilized on PES decreased when compared to the activity of the enzyme immobilized on PES–NH
2, which remained constant. These results demonstrated that the lipase enzyme showed better reusability on the PES–NH
2 membrane because of stronger attraction between the lipase enzyme and the support system
[37].
2.2.2. Immobilization on Solid Supports
The criteria for solid support used for enzyme immobilization include being nontoxic, safe for the environment, inert, inexpensive, and capable of withstanding microbial attack and degradation. The solid support protects the immobilized enzyme from harsh conditions during the reaction
[12][39]. All these factors, when present in solid support, enhance the reusability of the immobilized enzyme.
Figure 2 shows examples of various enzymes immobilized on different solid supports and their applications.
Figure 2. Examples of enzyme immobilization on various solid supports and their applications
[34].
2.3. Cost
Enzymes are valuable industrial biocatalysts that have been applied in a wide range of processing and manufacturing industries
[40]. The global market for industrial enzymes is projected to climb to USD 7 billion by 2023, from approximately USD 5.5 billion in 2018, with a compound annual growth rate of 4.9%
[41]. This could be because industrial enzymes are considered a significant alternative to conventional chemical catalysts due to the attractive advantages they offer, including their accessibility from renewable resources, substrate and product stereochemistry selectivity, and fewer subsidiary reactions, and thus fewer waste byproducts. In addition, industrial enzymes show better catalytic efficiency than normally applied catalysts under mild pH and temperature conditions
[40][42]. To date, there is limited economic analysis of enzyme-based biocatalysts, and thus, the cost-effectiveness of enzymes continues to be an intensively debated topic in industrial applications, particularly due to the harsh conditions that normally occur, namely, high pressures and temperatures, low and high pH, and oxidative environments. These conditions can easily inactivate enzymes; therefore, it is necessary to enhance the performance of these biocatalysts under the required operational conditions by improving their stability. This will increase the cost-effectiveness of their industrial implementation
[25][40]. Improvements can be made using genetic engineering and recombinant DNA or immobilization technologies. In considering these technologies, it is essential to understand the overall cost and sustainability of each technique, which can be done through life cycle assessments (LCAs). Taking into consideration the chemical inputs, energy consumption, and harmful outputs, LCAs evaluate the net environmental impact of all the steps in the industrial process. Technoeconomic analysis can be used in combination with LCAs to assess the ability to apply biocatalytic techniques in large-scale processes
[43][44][45].
2.4. Scaling-Up and Bioreactors
A bioreactor refers to a system that supports a biological process where a biochemical substance (enzymes, bacteria, etc.) are used. Different bioreactors have been employed for multiple applications, including the elimination of pollutants. When designing a bioreactor, two things must be considered. First, substantial research must be carried out on the biological system being used. Second, it is important to recognize the different parameters that need to be controlled, such as capital costs, installation and maintenance costs, stability, and scale-up
[46][47].
The idea of enzyme immobilization reactors is based on the immobilization of the enzyme into a support ionic interaction or covalent bonds. There are several criteria to address before constructing an enzyme bioreactor. The catalytic activity of the enzyme should be at the highest possible level in regard to the units of enzyme per gram of support. Additionally, the membrane or the support used should have a second purpose. For example, it could be used to separate products and substrates. The material should be inert and have no interaction with the products and substrates, and it should provide excellent mechanical resistance. It is important to decide when the immobilized enzyme will be replaced after multiple reaction cycles. This is an important decision because it will affect the cost of production. Usually, enzymes in industrial applications will be replaced when they reach 50 to 10% of their original activity. Another essential criterion is the reaction temperature because temperature has a large impact on kinetics. Typically, immobilized enzymes have better stability over a larger range of temperatures. Last, the process needs to be simple, easy, and inexpensive
[48][49]. Three main types of bioreactors can be scaled-up for enzyme immobilization on an industry-level: fixed bed reactors or packed column reactors, fluidized bed reactors, and stirred tank reactors.
Figure 3 illustrates these three different bioreactors. Choosing the best option for industrial application, such as water remediation and the removal of harmful toxic emerging pollutants, will depend on the reaction kinetics and the type of support used
[49].
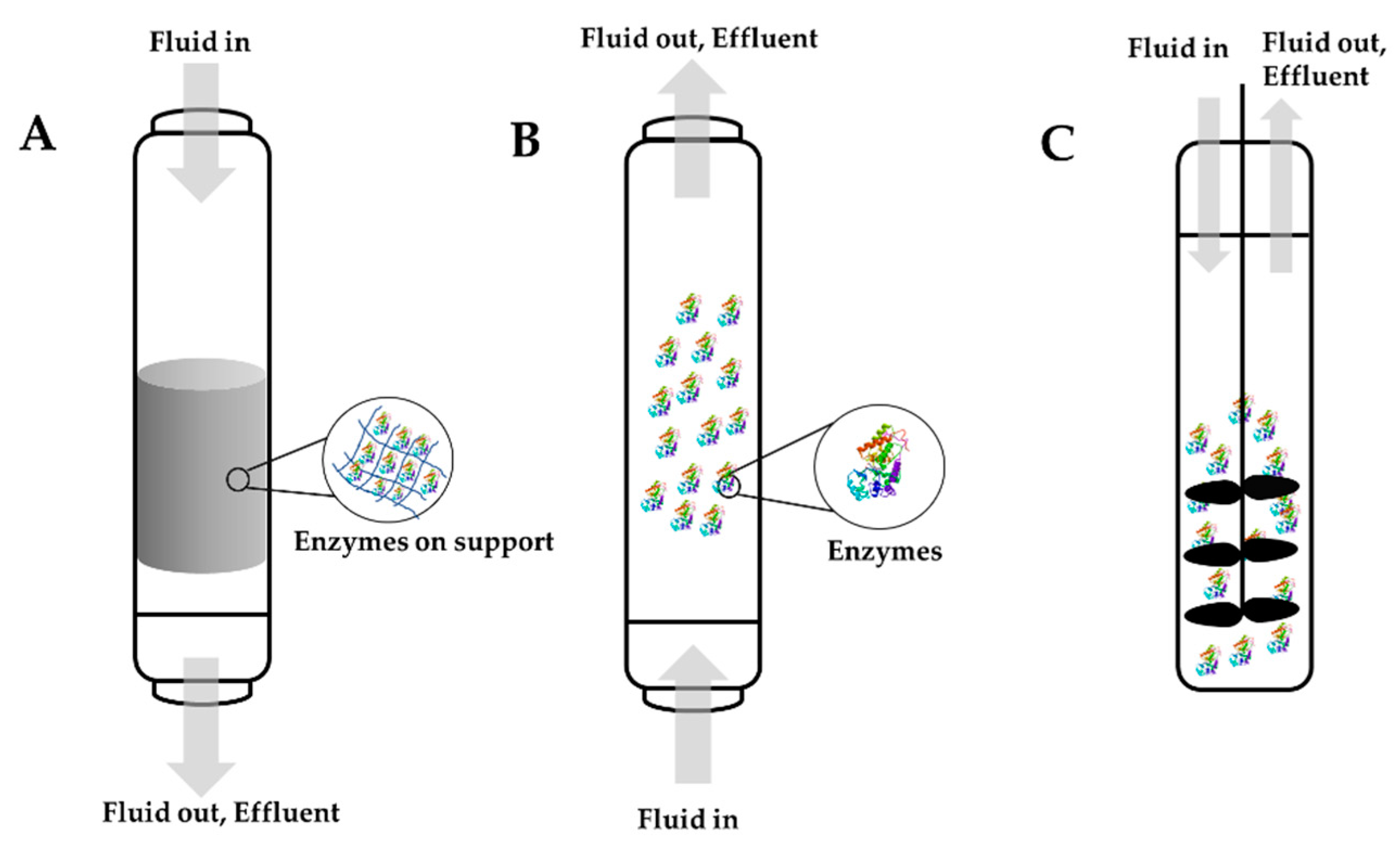
Figure 3. Some of the commonly used types of bioreactors: (A) fixed bed bioreactors, (B) fluidized bed bioreactors, and (C) stirred tank bioreactors.
3. Conclusions
Although promising, enzyme-based remediation approaches have many major challenges that still need to be addressed. Recent progress focusing on oxidoreductases to make enzymatic wastewater treatment processes and their applications were discussed and summarized. Efficient immobilization of enzymes can circumvent some of these challenges by enhancing the stability of many enzymes and increasing the operational pH and temperature ranges. Nevertheless, there remains a need for additional research to develop new and/or hybrid materials that can address some of the drawbacks associated with currently available supports. In addition, there is still a large gap between lab level work, field research, and the scaling-up/bioreactor application for these enzymes. Future research should focus more on the real-life application of using enzymes in existing wastewater treatment plants.
This entry is adapted from the peer-reviewed paper 10.3390/nano11113124