2. Development and Findings
Figure 1. (a) Schematic drawing (cross-section, not in scale) of the microfluidic device used in this study. Some of microchambers held a single cell inside, by pressing down the soft porous PDMS chip onto the cell culture solution placed on the glass of the dish. The glass-bottom dish was then filled with pure water to prevent the microchambers from drying. The cells in the closed microchambers can survive three days or more. (b) A representative image of cells in confinement in our microfluidic device, observed with an objective lens of 4X. Numbers in the figure show the number of cells confined in each microchamber, and arrows indicate the cells. Scale bar, 500 μm.
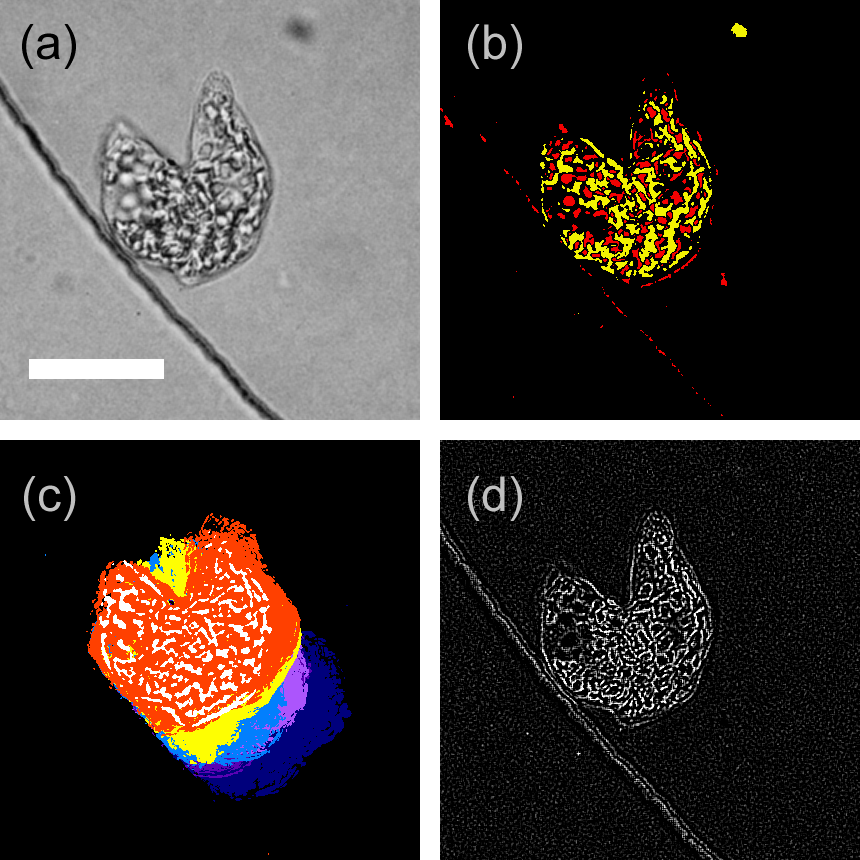
Figure 2. Examples of image processing for cell tracking, wherein a cell in the later stage of cell division at the edge line of circular microchamber was processed. (a) Captured image; obtained by 3 × 3 mean filtering with gray scaling. (b) Contrast image; obtained by thresholding brightness deviated from whole area average, i.e., the brighter part is colored in red, whereas the darker part is colored in yellow. (c) Trace image; obtained by time differential (frame-by-frame differential for each pixel) of the captured image, with further processing of binarization and integration for 20 frames (5.3 s). Five trace images were superimposed with different colors for effective visualization. (d) Focusing image; obtained by the spatial derivative for 3 × 3 pixels on the captured image. Scale bar, 20 μm.
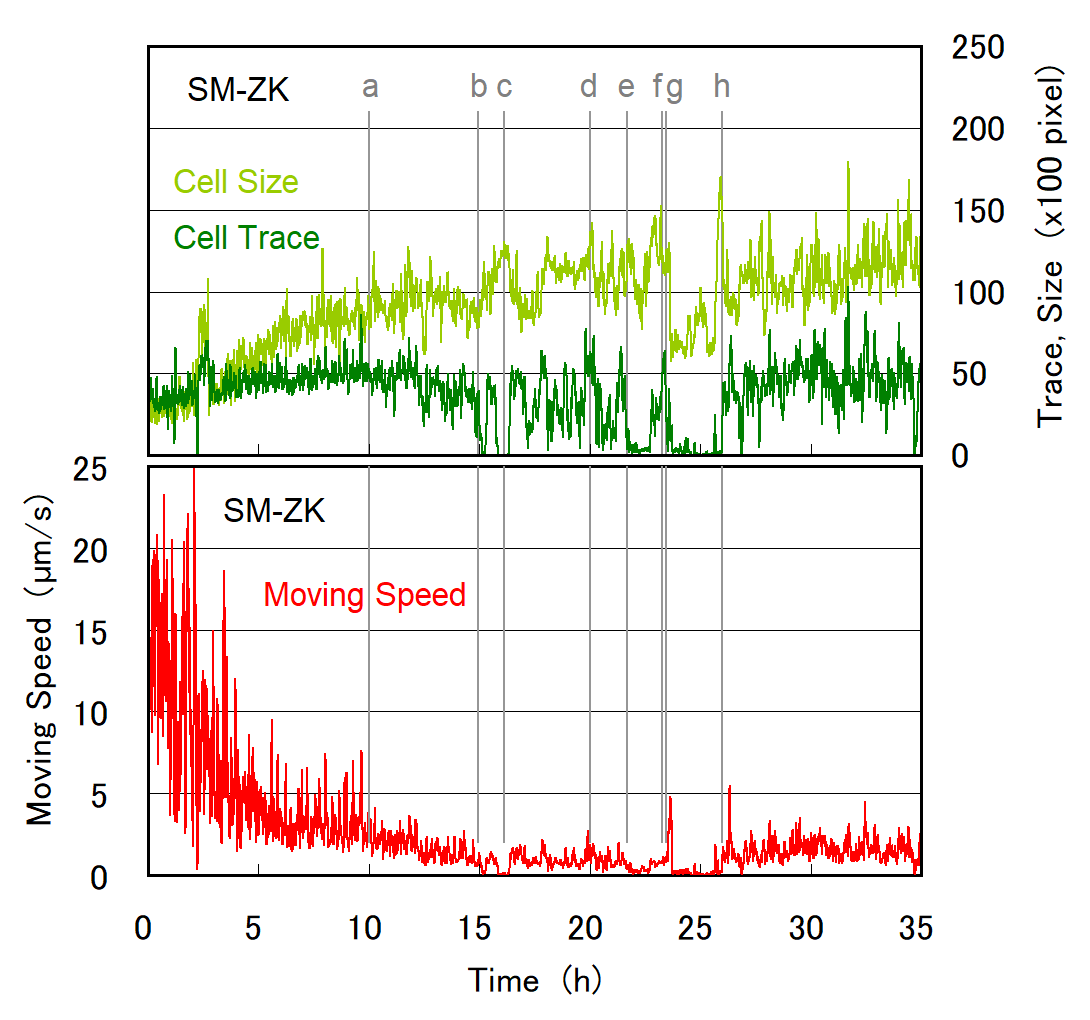
Figure 3. Temporal evolution of moving speed (velocity), cell size, and trace value obtained during the time course of cell division for an SM-ZK cell. Moving speed was calculated from XY-stage movement and the center of the gravity of the contrast image (e.g., Figure 2b). Cell size was obtained by counting red/yellow pixels in each contrast image (e.g., Figure 2b). The cell size represents the quasi-2D (10 μm thick) volume of the cell, assuming that cell height is at a uniform height of 10 μm. Trace value was obtained by estimating the non-zero pixels (white and red) in the trace image (e.g., Figure 2c). The trace value represents the momentum of cell movement and activity inside the cell. When the cell stops moving, the moving speed drops to zero, whereas the trace value may not become zero if the organelles move inside the cell. All of three values in Figure 3 were time-averaged for 2 min. The contrast and trace images for (a) to (h), and video-recorded images are given in Figure 4 and Figure 5, respectively.
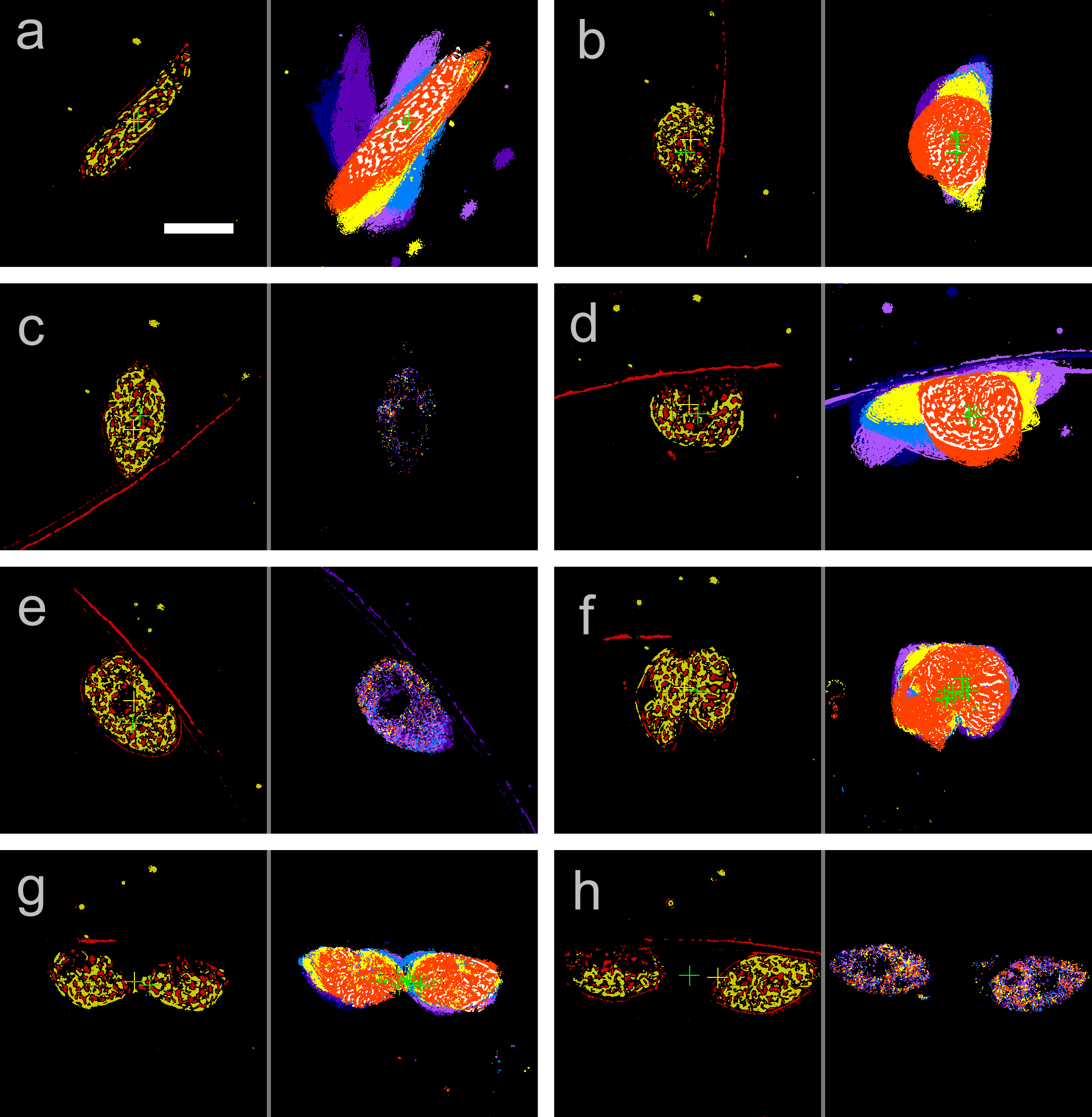
Figure 4. Contrast (left) and trace image (right) observed at (a) 10.0 h, (b) 15.0 h, (c) 16.1 h, (d) 20.0 h, (e) 21.7 h, (f) 23.3 h, (g) 23.5 h, and (h) 26.0 h. The time point of each image is given in Figure 3 as vertical lines. Cross marks in the images are the center of gravity of non-zero pixels for the purpose of XY-stage control. Scale bar, 20 μm.
Figure 5. Images extracted from the video recording of the experiment depicted in
Figure 3. (
a) 21.24 h, (
b) 21.60 h, (
c) 21.72 h, (
d) 21.75 h, (
e) 21.83 h, (
f) 21.92 h, (
g) 22.08 h, (
h) 22.17 h, (
i) 22.30 h, (
j) 22.58 h, (
k) 22.73 h, (
l) 22.92 h, (
m) 23.08 h, (
n) 23.28 h, (
o) 23.32 h, and (
p) 23.46 h. Time index given in the figure is shifted as reference; 00:00 (hh:mm) corresponds to 21.24 h in
Figure 3. The eyespot indicated by arrow is enlarged by four times in the inset. Scale bar, 20 μm. Dynamic evolution of cell division is provided in the
supplementary movie S3.
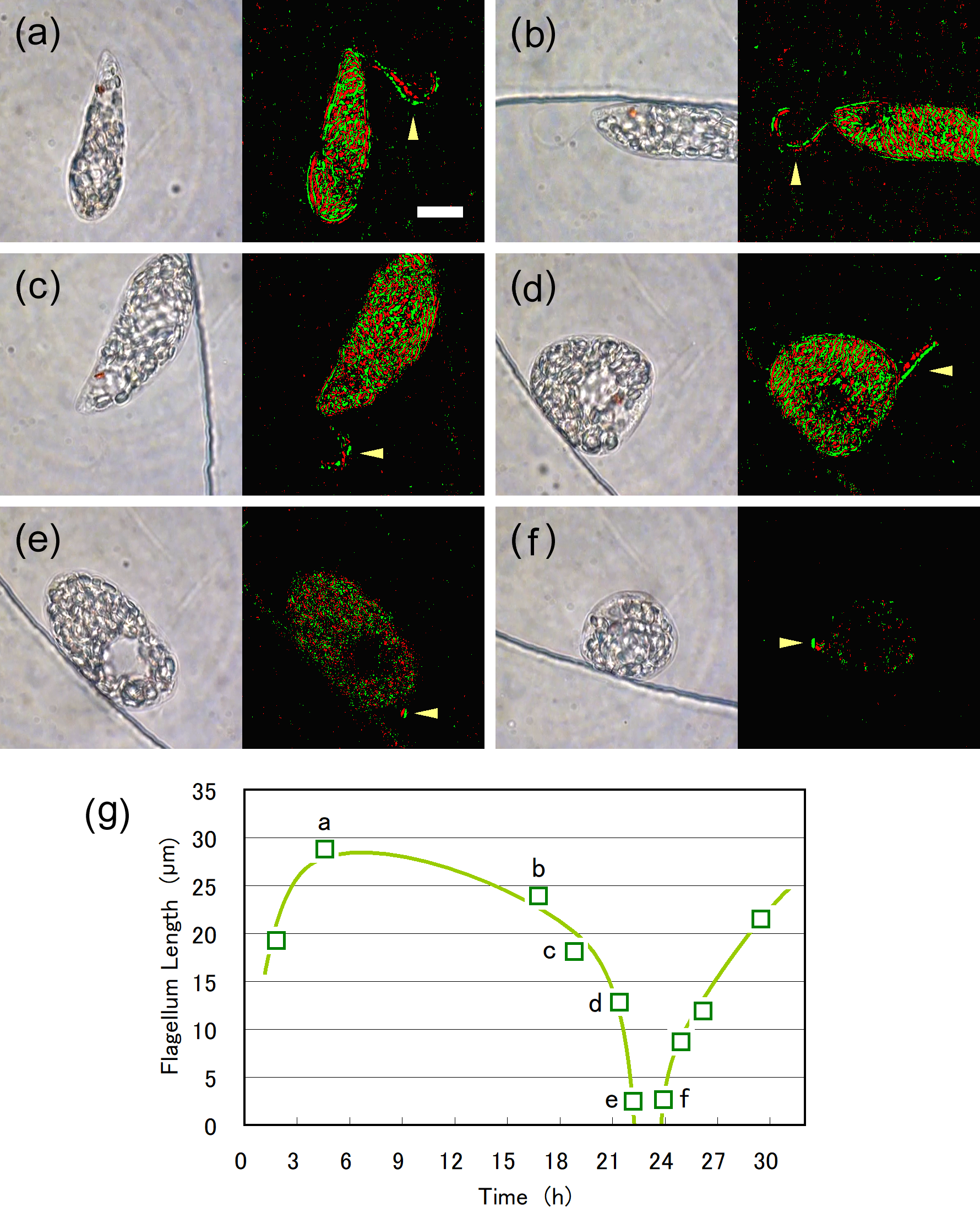
Figure 6. Shortening of the emergent flagellum, as seen in the images extracted from the video recording (left panels) and enhanced images (right panels) of the Figure 5 case. Enhanced images were obtained with differentiation and binarization of two images at 61 ms intervals. The length of the emergent flagellum was (a) 29 μm at 4.57 h, (b) 24 μm at 16.78 h, (c) 18 μm at 18.83 h, (d) 13 μm at 21.41 h, (e) 2.5 μm at 22.18 h. (f) After the cell division, the emergent flagellum (2.6 μm in length) was first observed for one of the daughter cells at 23.91 h. Scale bar, 10 μm. (g) Temporal change in flagellum length. The graphical line is provided to indicate the trend.
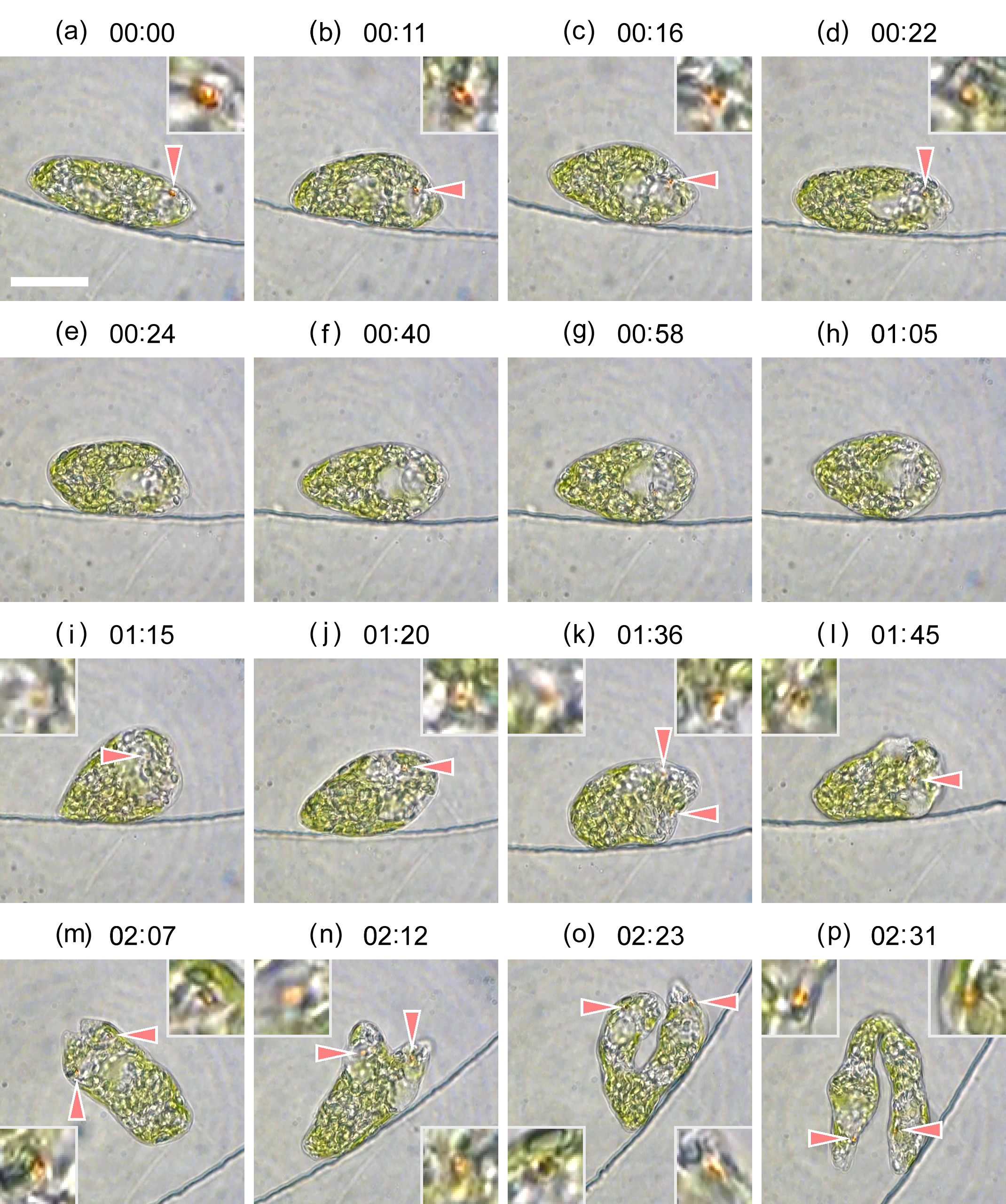
Figure 7. Images extracted from the video recording of the experiment depicted in
Figure S1. (
a) 17.52 h, (
b) 17.70 h, (
c) 17.79 h, (
d) 17.89 h, (
e) 17.92 h, (
f) 18.19 h, (
g) 18.49 h, (
h) 18.60 h, (
i) 18.77 h, (
j) 18.85 h, (
k) 19.12 h, (
l) 19.27 h, (
m) 19.64 h, (
n) 19.72 h, (
o) 19.90 h, and (
p) 20.04 h. Time index given in the figure is shifted as a reference; 00:00 (hh:mm) corresponds to 17.52 h in
Figure S1. The eyespot indicated by arrow is enlarged by four times in the inset. Scale bar, 20 μm. Dynamic evolution of cell division is provided in the
supplementary movie S4.
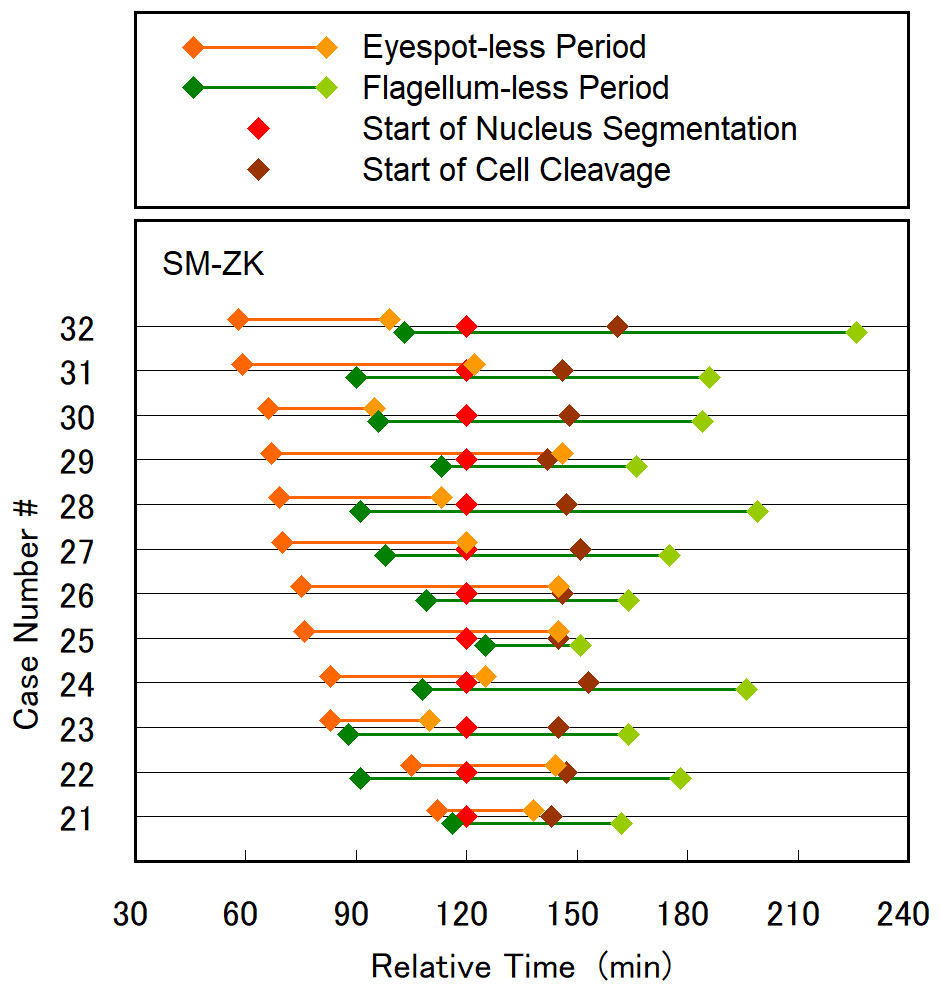
Figure 8. The period of disappearance of the eyespot and flagellum plotted for 12 SM-ZK cells, together with the initiation time of nucleus segmentation and cell cleavage. In the plot, the initiation time of nucleus segmentation was used as the standard timing (120 min). Case number was sorted by the time of eyespot disappearance (earlier to later). The case #30 corresponds to
Figure 3,
Figure 5 and
Figure 6, and
Movie S3. See
Figure S5 for WT cells.
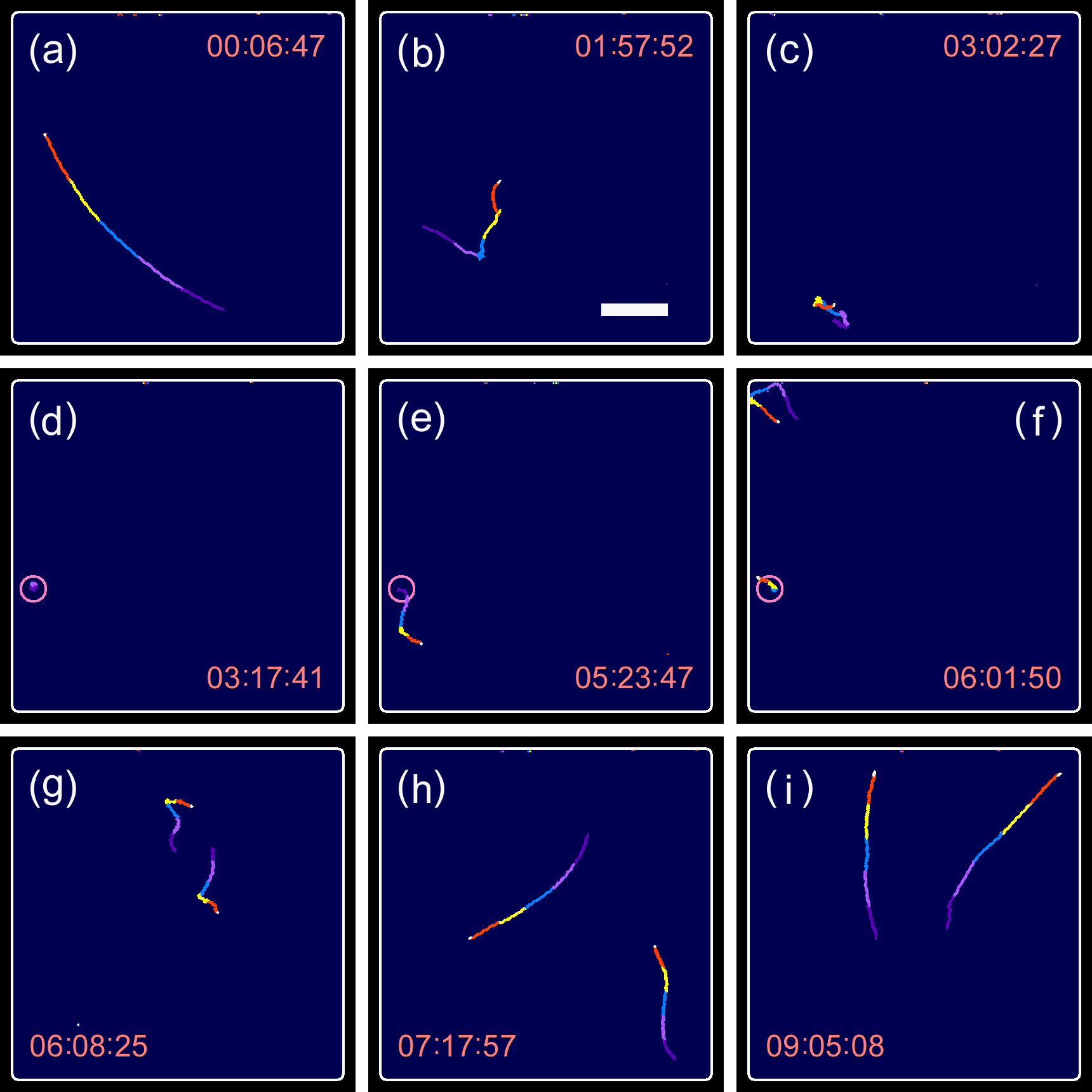
Figure 9. Swimming traces observed in a long-term millimeter-scale observation, including the period of cell division. (
a–
d) Movements of the parental cell, (
e–
i) movements of the daughter cells. In the images shown in (
a–
i), the traces obtained for the last 12.3 s (5 frames × 2.47 s/frame) were superimposed with different colors for visualization. Circles in (
d–
f) show the location where the cell division occurred. Refer to
supplementary movie S6 for visualizing the entire process. Time index in the panels is indicated by hh:mm:ss. Scale bar, 500 μm.
Figure 10. Histogram of the duration of cell division (non-swimming period) and difference in swimming start time between two daughter cells, based on data obtained from 26 individual SM-ZK cell divisions. The average cell division duration and difference in swimming start time was 157 and 38 min, respectively.
Although there are several studies on the cell division of
E. gracilis, a majority of them focus on nucleus segmentation with a few describing eyespot behavior. Buetow reviewed the morphology and ultrastructure of
Euglena [1] and reported that the stigma of
E. gracilis is composed of many spherical granules (diameter of 100–300 nm), which are present in the cytoplasm in a loosely packed group
[11][12]. The review also pointed out that the eyespot is a functionally specialized organelle, and that it is self-reproducing and occupies a defined portion of the cytoplasm. Leedale analyzed the mitotic cycle in live
E. gracilis cells under an optical microscope, and showed that the locomotor apparatus (flagella, photoreceptor, and eyespot) replicates and the reservoir divides during early-to-late metaphase succession
[13]. Hall and Jahn
[14][15], and also Gojdics separately
[16], reported that eyespot breaks up into its component granules during cell division at the stage of flagellum duplication or nucleus migration, with optical microscopy, although no evidential image showing eyespot break up was presented in their reports. Gillott and Triemer reported on the ultrastructure of
E. gracilis at different stages of mitosis from preprophase to telophase, as observed under an electron microscope
[17]. The only remark on the flagellum or eyespot found in the literature was that four non-emergent flagellar cross-sections were observed in a cell at prophase. Based on electron micrographs, Kivic and Vesk revealed that the eyespot replicated after nuclear division and segregated to opposite sides of the reservoir at about the same time as the flagella duplicated
[18][19]. These observations and findings were, however, rather fragmented and non-qualitative, and observed in fewer cases. The dynamic live cell imaging of
E. gracilis cell division in the present study will offer insight into the replication processes of the eyespot and flagellum, as well as the mechanisms required for their reproduction.
Dynamic live cell imaging of cell division revealed that the eyespot of
E. gracilis is removed/discarded once during cell division by a gradual shrinking process, or possibly the carotenoids in the eyespot are extracted/decomposed. We have not observed the break up of eyespot into a number of small globules in our dynamic live cell imaging of cell division, as suggested by the former literature
[14][15][16]. On the other hand, Kato et al. reported that their genetically modified (Eg
crtB-suppressed) colorless strain of
E. gracilis had no obvious red eyespot but still possessed empty eyespot globules
[20]. The report implies that the eyespot reproduction proceeds in two steps; the membranes of eyespot globules are reproduced, and then carotenoids accumulate into the globules.
Figure S7a shows an example before the reappearance of the new distinctively discernible eyespot (after nucleus segmentation and before cell cleavage), in which a reddish brown hazy region can be seen between the two nuclei. This may present newly synthesized carotenoids, which will eventually be loaded into the eyespot globules.
The shortening of the emergent flagellum of
E. gracilis is also the key for motility during cell division, i.e., the cell cannot swim due to the lack of emergent flagellum. The remaining issue in the flagellum shortening is whether the flagellum is decomposed from its outer end point or is retracted inside the reservoir from the backend. During the shortening process, a white dim spot was often observed at the outer end point of the flagellum (as shown in
Figure S7b) even for extremely short flagellum of almost zero length. Although the origin of the white spot has not been identified, our observation suggests that the flagellum is retracted inside the reservoir, whereas the outer end point remains unchanged. The retraction of emergent flagellum during cell division has been also reported for
C. reinhardtii [21].
The reason why
E. gracilis degrades the eyespot and flagellum and regenerates them (instead of generating a new organelle and distributing new and old ones into two daughter cells) still remains enigmatic. We consider that the organellar decomposition/reproduction function of the organism is more basic and advantageous than that of division and distribution. Degradation of organelles is a basic function of cells and is required for the removal of unnecessary and defective cytosolic components. Regeneration of organelles is also frequently observed in microbes, as evidenced by the observation that
E. gracilis cells regenerate their flagella once it is lost by external stimuli
[22][23]. Beech et al. reviewed instances of flagellum duplication inside the basal body of algal flagellates, which included observations of flagellum shortening (
Epipyxis) or complete retraction (
Chlamydomonas,
Pleurochrysis) during mitosis
[24].
Our observation revealed that each of the two daughter cells of
E. gracilis possess a newly replicated eyespot and emergent flagellum, and therefore the life span of
E. gracilis may be “logically eternal.” At the same time, during the process of cell division shown in
Figure 5, the two new eyespots were not generated simultaneously. One eyespot appeared approximately 25 min before the appearance of the second one (
Figure 5i,k). Additionally, the two daughter cells seemed to grow at different rates after cell division, as evidenced by a relatively large difference in swimming start time (18 min on average,
Figure 10). These observations suggested that the two newly regenerated eyespot and flagellum may grow at different rates and mature at different timings, possibly due to unequal distribution of cytoplasm or stored nutrients in the two daughter cells during cell division. These differences produce diverse cellular characteristics of
E. gracilis, such as in their motile behavior of gravitaxis
[25], chemotaxis
[26], and phototaxis
[27][28][29]. Further investigation on cell division and maturity is required to explain this phenomenon in
E. gracilis and other algal flagellates.
WT and SM-ZK cells contain a number of large paramylon granules, which tend to hinder the detection of the eyespot and detailed tracking of the shrinking eyespot. Upon suppression of paramylon production in SM-ZK cells using RNA interference or genetic modification, the eyespot reproduction process can be elucidated in detail using optical microscopy. The carotenoid specie present in the eyespot globules has been identified by Tamaki et al. as zeaxanthin
[30]. Upon realization of fluorochrome-labeling of zeaxanthin molecules, fluorescence microscopy may be employed to track fluorochrome-labeled zeaxanthin molecules and analyze the replication process. Advanced laser processing method, such as eyespot destruction by focused femto-second laser pulses on live
E. gracilis cells
[31], is also a promising approach to investigate eyespot self-reproduction. A mutant
Euglena strain containing plastids but no eyespot may be generated when eyespot regeneration is eliminated by advanced laser processing.