Aqueous organic redox flow batteries (AORFBs) represent innovative and sustainable systems featuring decoupled energy capacity and power density; storing energy within organic redox-active materials. This design facilitates straightforward scalability, holding the potential for an affordable energy storage solution.
1. Introduction
The increasing energy demand, intensifying environmental issues, and the imperative for sustainability prompt the development of renewable energy, and will become the cornerstone of a modern energy system
[1]. However, the intermittent nature of renewables such as solar energy and wind makes their deep penetration into the energy system difficult. This challenge has provoked a pressing necessity for developing safe, efficient and inexpensive grid-scale energy storage systems
[2][3]. The most widely deployed grid-scale storage technology is pumped-storage hydropower (PSH), where the storage capacity accounts for over 90% of total global market
[4][5]. However, the PSH technology is limited by specific geographical conditions, low power density and elevated costs of installation
[6]. Thus, greater emphasis has been devoted to electrochemical energy storage systems with merits of geographical independence
[7], high energy density, low cost and high efficiency
[8][9]. Among various electrochemical energy storage systems, the redox flow battery (RFB) is a promising technology for a flexible, long life, and sustainable energy storage system
[10]. Compared to conventional solid-state or static batteries, the RFB cell stack is detached from the electrolyte reservoirs, thereby achieving the power-capacity decoupling
[11]. The power output is contingent upon the electrode area, whereas the capacity is governed through the concentration and magnitude of the electrolyte tanks
[12][13]. This merit offers the RFB not only scaling flexibility, but also great safety without thermal runaway. There are two types of RFBs based on solvent used, non-aqueous and aqueous. Even though non-aqueous RFBs have a high voltage range, they are facing unavoidable challenges, such as poor ion conductivity, flammability and toxicity relevant to organic solvents
[9][12]. Thus, aqueous RFBs with advantages of safety, cost-effectiveness and environmental friendliness are more attractive for applications involving extensive energy storage.
2. Aqueous Organic Redox Flow Batteries
During the past decade, there has been a substantial amount of attention and notable advancements in the field of AORFBs that utilize water-soluble organic compounds
[14]. Organic RMs offer several advantages, including abundant elements, reduced environmental footprint, and the potential for cost-effectiveness. The designability of these materials allow for precise tuning of their structures and chemical properties. For instance, the introduction of electron-donating (OH
−) can respectively shift the redox potential in the negative directions
[15]. Additionally, the incorporation of ammonium groups has been shown to enhance both solubility and electrochemical reaction rates. Furthermore, aqueous electrolytes, consisting of water, when used in conjunction with selective ion-conductive membranes offer numerous benefits, such as enhanced safety, cost-effectiveness, greater alignment with sustainability goals, and enhanced ionic conductivity, resulting in higher energy conversion efficiency. A key advantage, when compared to VRFBs, is that it exhibits superior reversibility in the redox processes and electron transfer rate.
In 2014, Aziz et al. proposed an anthraquinone-based AORFB, and it sparked research areas of keen interest in the field of RFBs
[16]. After that, numerous studies have focused on the advancement of innovative RMs within alkaline, acidic, or neutral pH solutions.
Figure 1 summarizes typical posolytes and negolytes under different pH conditions. In acidic conditions, anthraquinones (AQ) with potentials (0.1 V~0.3 V vs. SHE) are commonly used as negolytes while benzoquinones and phenothiazines (0.5 V~0.9 V vs. SHE) work as posolytes. In neutral or near neutral conditions, anthraquinones, phenazines, and viologens (−0.2 V~−0.6 V vs. SHE) are used as negolytes when ferrocenes and tetramethyl-1-piperidinyloxys (TEMPOs) (0.3 V~1.1 V vs. SHE) work as posolytes under mild pH conditions. In alkaline conditions, anthraquinones, benzoquinones and phenazines (−0.5 V~−0.8 V vs. SHE) are frequently selected as negolytes while few molecules are reported as posolytes.
Figure 1. Typical organic posolytes and negolytes under different pH conditions.
2.1. Quinone Derivatives
Aziz et al. reported the unprecedented AQ-based AORFB system in acid conditions
[16]. AQDS and Br
2 are employed as anolyte and catholyte in sulfuric acid solution. During charging and discharging, AQDS undergoes expeditious redox reaction (two-electron transfer) with a kinetic constant of 7.2 × 10
−3 cm/s, which demonstrates a notably faster rate than vanadium ions. The cell exhibits an open-circuit voltage (OCV) and achieves a peak power density of 0.92 V and approximately 1.4 W/cm
2 when it is at a 90% state of charge (SOC). At 10% SOC, the OCV decreases to 0.69 V, but the peak power density increases to around 3.3 W/cm
2.
2.2. TEMPO/Viologen Derivatives
Significant progress has been achieved concerning ORFBs operating in neutral aqueous media, utilizing (2,2,6,6-tetramethylpiperidin-1-yl) oxyl (TEMPO). TEMPO is widely used in organic posolytes, and undergoes a rapid redox reaction (one-electron transfer)
[17][18]. While being high, there is still room for substantial improvement to maximize the cell voltages in an aqueous RFB system well before reaching the point of oxygen evolution. However, TEMPO is insoluble in water. Liu et al. attached hydroxyl groups to the 4-positions yielding 4-OH-TEMPO, which exhibits a solubility of 2.1 M in water
[19]. Through Equation (1)
[20], it has been determined that the kinetic rate constant of 4-HO-TEMPO (2.4 × 10
−3 cm s
−1) is comparable to that of vanadium ions (5.3 × 10
−4 cm s
−1)
[21][22], where
i0 represents the exchange current,
F represents Faraday’s constant,
A represents the surface area of the working electrode,
n represents the number of transferred electrons, and
c represents the molar concentration. When employing methyl viologen (MV), the battery demonstrates an exceptionally elevated cell-voltage, measuring 1.25 V, and consistently maintains its capacity over 100 cycles with an almost perfect Coulombic efficiency. However, the degradation of 4-OH-TEMPO does exist, particularly under acidic or alkaline conditions. This is attributed to the self-oxidation of the hydroxyl groups at the 4-position by the oxidized 4-OH-TEMPO. Prior to electrochemical charging, TEMPOL
+ may form under acidic conditions via a chemical reaction. At higher OH
− concentrations, it can chemically convert back to TEMPO, rendering TEMPO irreversible electrochemically in strongly alkaline electrolytes. This suggests that TEMPO should be employed in aqueous solutions close to neutral pH to avoid side reactions
[20].
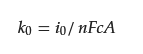
To suppress such degradation of 4-OH-TEMPO, Janoschka et al. replaced OH with quaternary ammonium groups, and combined this with methyl viologen (MV),which demonstrated a remarkable capacity retention of almost 100% over 100 consecutive cycles, suggesting the robust electrochemical stability of N,N,N-2,2,6,6-heptamethyl piperidinyl oxy-4-ammonium chloride (TEMPTMA)
[23]. TEMPTMA exhibits an impressive solubility level, reaching 3.2 M in 0.3 M NaCl, which corresponds to a capacity of 85 Ah/L, respectively.
2.3. Ferrocene Derivatives
Ferrocene derivatives are highly redox-reversible molecules composed of two cyclopentadiene molecules with Fe-ion in the center
[24][25]. The iron center undergoes a reversible one-electron transfer process, yielding a redox potential approximately 0.4 V. Thus, making ferrocene derivatives suitable as catholytes for AORFBs
[26][27]. Nevertheless, ferrocene is hard to dissolve in water, hindering its direct application in AORFBs
[28]. Thus, considerable dedication has been devoted to make water-soluble ferrocene derivatives. Liu et al. proposed water-soluble derivatives of ferrocene unprecedentedly by modifying ferrocene with quaternary ammonium groups, namely (ferrocenylmethyl) trimethylammonium chloride (FcNCl) and N
1-ferrocenylmethyl-N
1,N
1,N
2,N
2,N
2-pentamethylpropane-1,2-diaminium dibromide (FcN
2Br
2)
[29]. By introducing the hydrophilic functional groups, solubility of FcNCl in water can reach 4. 0 M, while that of FcN
2Br
2 is 3.1 M. Therefore, greatly enhancing the capacity of ferrocene derivatives. The battery consisting of FcNCl/methyl viologen delivers an OCV and power density of 1.06 V and approximately 125 mW/cm
2. Furthermore, the theoretical energy density of this particular battery is up to 45.5 Wh/L. At 0.5 M, the FcNCl/MV cell exhibited a daily capacity degradation rate of 0.58%.
2.4. Challenges of Conventional AORFBs
Despite a series of pioneering studies demonstrating favorable electrochemical performance, the advancement of AORFBs still presents numerous known and unknown challenges. Firstly, the energy density of AORFBs has not yet exceeded that of their competing VRFBs. This discrepancy can be attributed to the comparatively lower solubility and electrochemical potential of organic RMs in comparison to VRFBs. The limited solubility of organic RMs (such as quinone, TEMPO, fluorenone, viologen, phenazine, and alloxazine) poses a significant obstacle to enhancing energy density. While numerous promising organic RMs have been created for AORFBs, the majority have only been tested at restricted concentrations (below 0.5 M). Secondly, the potential for crossover of the electrolytes still exists, which can result in a decline in capacity and energy, thereby diminishing the lifetime of the AORFB system. Lastly, the observed capacity degradation-rate falls short of the stability criteria necessary for large-scale applications.
3. Evolution of Redox-Targeting Based System
3.1. Concept and Principles
The concept of redox-targeting (RT) was first introduced in 2006 to address the solubility issues encountered in the AORFB system. This concept originates from both molecular wiring and polymer wiring, such as in the case of LiFePO
4. LiFePO
4 itself exhibits poor electronic conductivity, necessitating the incorporation of a substantial amount of carbon black to form a continuous conductive network for electron penetration. However, the inclusion of a large quantity of non-active conductive agents significantly diminishes the power density of the system. Therefore, Wang et al. sought to introduce a chemisorbed monolayer of redox-active molecules on its surface, attempting molecular wiring of the battery material
[30].
The RM undergoes electrochemical reactions on the electrode, resulting in its oxidation or reduction. Subsequently, it enters into chemical reactions with the solid materials within the tank, eliminating the need for direct electrode contact
[30]. Moreover, solid materials are often configured in a granular form due to the advantages of rapid charging rates and facilitating dense packing. The chemical reactions between the solid materials and RMs occur within the tank. Consequently, the design of the tank size directly impacts the utilization efficiency and reaction rate between them. Therefore, the tank needs to meet three essential requirements.
To increase energy density, three strategies can be employed: (1) increasing the battery voltage, (2) enhancing the actual concentration of RMs, and (3) achieving multiple redox processes
[31]. Despite the potential for non-aqueous systems to offer higher cell voltage, thereby increasing their energy density, it is worth noting that these systems tend to exhibit relatively lower ionic conductivity for organic electrolytes. In contrast, aqueous electrolyte systems, while yielding lower voltage due to hydrogen and oxygen evolution reactions, possess excellent ionic conductivity along with enhanced safety, economic viability, and environmental benefits
[14].
3.2. Two-Molecule RT Reaction
In the initial exposition of the RT reaction, it was a common practice to utilize two distinct RMs possessing appropriate potentials, which were paired with a single active solid material. This pairing was performed with the objective of facilitating two-way RT reactions. In 2013, Huang et al. proposed the pioneering redox-flow lithium battery (RFLB), which was a cathodic half-cell. This innovation operated within the cathodic tank, utilizing LiFePO
4 as the solid material, ferrocene, and 1,1’-dibromoferrocene as the RMs in the catholyte
[32]. The feasibility of RFLB was substantiated through experimental results and various characterization techniques, which provided conclusive evidence of the occurrence of redox-targeting in the system. In 2014, Pan et al. constructed an assembly of an anodic half-cell of RFLB with CoCp
2 and CoCp*
2 as RMs and using TiO
2 as the solid materials
[33].
Fan et al. showed the operation of lithium polysulfide cells in both continuous and intermittent flow modes unprecedentedly
[34]. However, excessive conductive additives can increase viscosity, reducing energy storage capacity. Moreover, as the concentration of RM increases, the physical and electrochemical properties of RM may deteriorate. In order to tackle the mentioned challenges, Li et al. implemented the “redox-targeting” concept in such flow batteries, which utilized two RMs, CrCp*
2 and NiCp*
2 with high stability to achieve the reversible lithiation/delithiation of S/Li
2S
[35].
Recently, Hatakeyama-Sato et al. presented 4 V-class organic polymer RMs that incorporate thianthrene derivatives, which exhibit higher potentials compared to conventional organic RMs
[36]. This increased potential allows for the charging of LiMn
2O
4, with a significant theoretical capacity of 500 Ah/L. The design of soluble or nanoparticle polymers proves useful in mitigating the crossover, with only approximately 3% observed after 12 days of testing, while also contributing to mediating reactions. By developing thianthrene-based RMs with distinct molecular geometries, including small molecules, they also found that the polymeric materials with larger molecular radii had a positive impact on mitigating undesired crossover reactions, even porous separators. These findings hold the promise of advancing the development of flow cells with an organic/inorganic hybrid design, resulting in flow cells that offer higher energy density and extended lifespan. The design of this polymer is aimed at preventing quick permeability. The reason is that the monomer molecules have a small diameter, which leads to rapid penetration. The polymer does not affect the redox potential. However, a drawback is that the solubility of the polymer is low, causing an RT reaction to occur between solid materials and particles. This leads to a reduction in diffusion rate and a decrease in the contact area between them.
3.3. Single-Molecule RT Reaction
Nonetheless, the two-molecule RT reaction typically results in a voltage decline due to the relatively substantial disparity in their potentials. Furthermore, during a comprehensive cell demonstration, the two-molecule system encompasses the involvement of four distinct RMs and undergoes a total of four successive stages of reactions. This intricate setup introduces significant operational complexity and adversely impacts the cycling stability of the battery
[37]. To mitigate these concerns, Zhou et al. first introduced the concept of single-molecule redox-targeting (SMRT) instead of two RMs, which resulted in the attainment of a remarkable 95% voltage efficiency with the molecule (1-Ferrocenylmethyl-3-methylimidazolium bis (trifluoromethanesulfonyl) amide, FcIL)
[38]. This achievement also led to a considerable upsurge in energy density to 330 Wh/L without compromising the essential characteristics of RT-AORFBs and simplifies the composition of the electrolyte. The reaction between RM and the solid materials relies solely on the Nernstian potential difference, which makes the voltage profile demonstrate a single charge/discharge plateau, effectively eradicating voltage hysteresis and thereby significantly enhancing voltage efficiency.
In 2018, Yu et al. employed [Fe(CN)
6]
4−/[Fe(CN)
6]
3− and S
2−/S
22− as RMs in the catholyte and anolyte. The cell achieved an impressive volumetric capacity
[39]. Upon loading LiFePO
4 and LiTi
2 (PO
4)
3 as the solid materials into the cathode and anode sides, it attained an anodic capacity of 305 Ah/L and a cathodic capacity of 207 Ah/L, which outperformed the VRFB, exhibiting a 4- to 6-fold improvement. Chen et al. employed Prussian blue (Fe
4[Fe(CN)
6]
3, PB) as a cost-effective and robust capacity-enhancing material for utilization in neutral aqueous RFBs in 2019
[40]. The introduction of PB as a solid material in the system, coupled with its chemical reactions with [Fe(CN)
6]
4−/3−, has effectively overcome the solubility limitations. This breakthrough has significantly enhanced the electrolyte’s capacity. Compared to other [Fe(CN)
6]
4−/3− systems, this electrolyte configuration has achieved an unprecedented volumetric capacity of 61. 6 Ah/L, setting a remarkable record.
4. Redox-Targeting Based RT Systems
4.1. Anthraquinone (AQ)-Based RT Systems
Conventional RFBs with inorganic species often employ transition metal ions under acidic condition. However, these systems encounter several obstacles, such as high material costs, crossover issues, and the use of corrosive or hazardous electrolytes, which impede their large-scale deployment. In contrast, the use of organic RMs abundant in nature has emerged as an appealing alternative to inorganic counterparts in RFBs
[41]. Nonetheless, these systems still grapple with the persistent challenge of low solubility of RMs. To mitigate the low solubility issue of 9,10-anthraquinone-2,7-disulphonic acid (2,7-AQDS) under neutral conditions, Zhou et. al. employed the SMRT reaction, utilizing cost-effective polyimide as the solid material, which enhanced its capacity to 97 Ah/L
[42]. Thanks to the structural similarity between polyimide and AQDS, as well as their identical redox potentials, the energy density of the cell was improved to 39 Wh/L, with polyimide boosting the capacity of 2,7-AQDS in a neutral buffer solution by over eight times.
4.2. TEMPO-Based RT Systems
Schröter et. al. presented the first investigation of a fully organic Nernstian potential-driven RT system, comprised of poly (TEMPO-methacrylate) (PTMA) as the solid materials and N,N,N-2,2,6,6-heptamethylpiperidinyloxy-4-ammonium chloride (TMATEMPO) as the single RM, which reached a capacity utilization of 78% of solid materials
[37]. After the addition of PTMA, the capacity of the battery increased from 3.7 mAh to 11.4 mAh. However, originally, PTMA was hydrophobic, but as the cycling progressed, it gradually became hydrophilic. This change also led to material swelling, resulting in increased internal pressure and consequently, electrolyte leakage. For the PTMA/TMATEMPO system, the increasing pressure by the PTMA swelling resulted in recurrent cell failures around the 50-cycle mark due to electrolyte leakage. Such expansion not only reduces the battery’s capacity but also renders its volume and porosity unmeasurable. Consequently, rendering volumetric capacities unquantifiable. Therefore, TEMPO-based ORFBs still require further research efforts to address and improve the issue of material expansion. Despite the limitations mentioned, it showcased the potential of the all-organic RT approach and proposed future strategies to address the capacity and cyclability constraints. These strategies encompass enhancing the concentration of RMs, augmenting the interfacial area between RM and solid material, improving the hydrophilicity of the solid material, and enhancing the chemical compositions of RM and solid material, which will enhance the energy density and cycle-life of RT systems.
4.3. Ferrocene-Based RT Systems
In 2020, Yu et al. utilized Fc-SO
3Na, a sulfonated ferrocene derivative, as the cathodic RMs and zinc metal as the anode, which was designed for pH-neutral aqueous RFBs
[43]. Fc-SO
3Na boasts high water solubility and demonstrates impressive electrochemical reversibility and stability. This battery exhibits noteworthy characteristics at low concentration, including a capacity retention rate of 99.9975% per cycle. At higher concentrations it achieves an energy density of 27.1 Wh/L, which is competitively comparable to conventional VRFBs ranging from 25 to 30 Wh/L. Additionally, the introduction of LiFePO
4 as a solid material into the cathodic tank led to a RT reaction with Fc-SO
3Na and BrFc-SO
3Na. This resulted in a substantial increase in volumetric capacity, reaching 293. 5 Ah/L, assuming that the porosity of LiFePO
4 is 50%. Considering additional advantages such as excellent cycling stability, an eco-friendly pH-neutral supporting electrolyte, and the abundance of resources for the RMs and LiFePO
4 as the solid material, the RFB system holds tremendous potential for large-scale applications. After extended cycling, there has been a slight decline in the battery capacity attributed to the degradation of the zinc electrode as well as the occurrence of dendritic growth where metallic zinc tends to penetrate the membrane
[44].
4.4. Viologen-Based RT Systems
The preceding three organic species categories all operate under aqueous conditions, and it is worth noting that non-aqueous organic RT reactions based on viologen are also promising
[45]. Viologen, an anolyte, has gained widespread recognition in the fields of electrochemistry, primarily because of its stable 2+/1+ redox couple. Its counterparts have been extensively investigated as small molecules
[46][47], oligomers, or polymers, making them suitable for massive energy storage applications in both aqueous and non-aqueous environments. When it comes to RT-based flow battery (RTFB) applications, despite the initially promising nature of solid materials in granular form due to their high charge rates and accessible SOC, they caused issues like high backpressure and system clogging due to their tightly packed jagged shapes. To overcome these problems and maintain charging performance, Wong and Sevov synthesized spherical solid materials with polystyrene derivatives of viologen in a range of sizes through minor variations to water, benzene, and surfactant
[48].
4.5. Advanced Characterization Methods
Characterization methods are vital for understanding redox-active material properties and their operational and degradation mechanisms in RFBs. To formulate effective guidelines for designing RMs for RFBs, it is imperative to have a profound grasp of reaction mechanisms and cycling stability. Spectroscopic techniques, like ultraviolet and visible spectrophotometry (UV-Vis), X-ray photoelectron spectroscopy (XPS), X-ray diffraction (XRD) and electron paramagnetic resonance (EPR) spectroscopy, are preferred for studying RT reactions in the liquid electrolytes.
To monitor the changes in valence states of Na
3V
2(PO
4)
3 (NVP) in the cathode side, as well as the corresponding evolution of MPTZ concentration in real-time, operando X-ray absorption near edge structure (XANES) spectroscopy was employed to examine SMRT reaction
[49]. This technique is widely used to investigate variations in valence states and chemical environments in battery materials. When the SOC reached 60% for the mediators in the catholyte, there was minimal change in absorption. This suggests that the mediator is not effectively oxidizing NVP in this range, and the capacity is primarily contributed by MPTZ to MPTZ
+. However, when the SOC reached 65%, reaching a thermodynamic driving force threshold, the absorption edge of vanadium (V) rapidly moves in the direction of higher energy. This shift indicates significant changes in the valence state of V during the SMRT reaction. Notably, isosbestic points were observed at approximately 5495, 5518, and 5560 eV, indicating phase transitions of NVP with MPTZ
+ during SMRT reaction. These observations provide insights into the dynamic changes occurring in the system during the SMRT reaction and highlight the role of MPTZ
+ in the redox processes.
The EPR spectrum is obtained by measuring the sample’s absorption and radiation of microwave radiation. Fang et al. employed EPR to investigate a 1 mM TPz-1 sample prepared through electrochemical methods
[50]. The EPR spectrum reveals signals corresponding to the presence of free radical species in the sample. By analyzing the EPR spectrum, the redox mechanism of TPz-1 was determined, demonstrating the formation of a free-radical species during the charging process. This result further validates the hypothesis of TPz molecules possessing a six-electron redox-activity, making TPz a promising anode material for alkaline RFBs. However, TPz has a low diffusion coefficient and poor battery performance. The subsequent investigation can focus on studying more stable derivatives of TPz with an aim to increase their diffusion coefficient without compromising the redox-activity.
This entry is adapted from the peer-reviewed paper 10.3390/su152115635