1. Introduction
Direct cell–cell communication is mediated by gap junction channels that enable free exchange of small cytosolic molecules among neighboring cells. Each channel is formed by two hemichannels that create a hydrophilic passageway, spanning the plasma membranes of two neighboring cells and a narrow extracellular space (gap). A gap junction hemichannel is made of six radially arranged proteins named connexins in vertebrates and innexins in invertebrates. Connexins/innexins contain two extracellular lops (EL
1 and El
2) and three cytoplasmic domains: an NH
2-terminus (NT), a cytoplasmic loop (CL) and a COOH-terminus domain (CT; rev, in
[1]).
Gap junction channels are physiologically regulated by a chemical gating mechanism that is activated by changes in cytosolic ionic homeostasis, resulting from cell damage, inhibition of the metabolism, acidification and hypoxia, among others. Over the years, numerous studies have suggested that channel gating results from a rise in cytosolic calcium concentration ([Ca
2+]
i)
[2][3][4][2,3,4] in the high nanomolar–low micromolar range
[1][5][6][7][1,5,6,7]. Since the early 1980s,
rwe
searchers have proposed that Ca
2+i causes gating by activating calmodulin (CaM)
[1][7][8][9][10][1,7,8,9,10] via a cork-like pore-plugging mechanism
[11][12][13][11,12,13] probably involving conformational changes in connexins as well.
In the last four decades, many studies have reported that gap junction channels are sensitive to anesthetics. Channel gating induced by long-chain alcohols (heptanol and octanol) was first reported by Johnston and coworkers
[14] in crayfish septate axons. Soon after, gating by volatile anesthetics such as halothane and isoflurane was also reported in a variety of vertebrate and invertebrate systems
[15][16][15,16].
In spite of over four decades of research, the mechanism by which anesthetics cause gap junction channel gating is still poorly understood. Johnston and coworkers
[14] proposed an extracellular site of action for alkanols. Similarly, Eskandari and coworkers
[17] reported that, in inside-out patches of lens connexin hemichannels, the addition of 1 mM octanol did not affect the channel’s open probability or the unitary conductance, while in outside-out patches, addition of 1 mM octanol to the bath (extracellular surface of hemichannels) significantly reduced single-channel open probability without altering the unitary current. Therefore, they concluded that octanol inhibits lens connexin hemichannels by acting on a site accessible only from the extracellular space. However, the possibility that a soluble intermediate was washed away by the internal perfusion of crayfish axons
[14] and by the bathing solution of inside-out patches
[17] was not considered.
In 1991,
theour data seemed to also exclude the role of cytosolic Ca
2+ and pH in crayfish axons uncoupled by heptanol, halothane and isoflurane
[16]. However, based on the potentiating effect of caffeine and theophylline added to anesthetics, and the numerous studies published in the past three decades on the effect of anesthetics on both CaM’s Ca
2+ sensitivity and Ca
2+ release from the sarcoplasmic reticulum (SR) via inositol trisphosphate (IP3) and/or ryanodine receptor (RyR) channels, as well as evidence that ion-selective electrodes may be unreliable in the presence of anesthetics
[18],
reswe
archers are now reconsidering
theour earlier interpretation
[16]. As a result,
rwe
searchers propose an alternative hypothesis, that cell–cell uncoupling by anesthetics may result from CaM’s activation caused by anesthetic-induced [Ca
2+]
i rise, and increased Ca
2+ sensitivity in CaM.
2. Measurement of Junctional Resistance (Rj), [Ca2+]i and [H+]i in Crayfish Septate Axons
In
theour 1990s study
[16], crayfish axons were superfused with a standard saline solution for crayfish (SES)
[19], containing (in mM): NaCl, 205; KCl, 5.4; CaCl
2, 13.5 and 4-(2-hydroxyethyl)-1-piperazineethanesulfonic acid (HEPES), 5 (pH 7.5). Either 2.8–5.6 mM 1-heptanol, 9.5–28.5 mM halothane or 23.6 mM isoflurane was added to SES in the presence and absence of either 10–20 mM caffeine or 10-20 mM theophylline. For testing the effect of low pH
i [20][21][20,21], the axons were superfused with a sodium acetate saline solution (Ac) containing (in mM): Na acetate, 205, KCI, 5.4 and CaC1
2, 13.5 (pH 6.3).
Four microelectrodes were inserted into a lateral giant axon, two on each side of the septum (
Figure 1A), and hyperpolarizing square current pulses (150 nA, 300 ms) were passed every 10 s alternatively into the posterior (C
1) and anterior (C
2) axon segments. The resulting electrotonic potentials V
1 and V
2 (from current injection in C
1), V
1* and V
2* (from injection in C
2) and the membrane potentials (E
1 and E
2) were recorded with two voltage microelectrodes through a voltage follower. The voltage signals were displayed on an oscilloscope and a chart recorder, and were digitized. Both membrane (R
m1, R
m2) and junctional (Rj
1, Rj
2) resistances were calculated from current (I
1, I
2) and voltage (V
1,V
2,V
1*,V
2*) records (
Figure 1B)
[16].
Figure 1. Diagram of electrical recording setup (
A). Current pulses are injected via current microelectrodes (I
1, 1
2) alternatively in the posterior (C
1) and anterior (C
2) axon segments. The resulting potentials (V
1, V
2, V
1*, V
2*) are displayed in the chart recorder and the oscilloscope. The voltage signal (V
ion), recorded by the ion-selective microelectrode, is displayed in the chart recorder after subtraction of membrane potential (V
m). (
B). Equivalent circuit and equations used to calculate junctional (Rj
1, Rj
2) and non-junctional (R
m1, R
m2) resistances. Adapted from
[21].
[Ca
2+]
i and [H
+]
i were measured with ion-sensitive microelectrodes based on neutral-carrier sensors. Ca
2+ microelectrodes used the calcium cocktail (ETH 129) and H
+ microelectrodes used the proton cocktail tri-n-dodecylamine. At the time,
rwe
searchers thought that these ion-selective microelectrodes were insensitive to anesthetics. Later on, however, ion-selective microelectrodes were proven unreliable in the presence of anesthetics
[18]. In contrast, these ion-selective microelectrodes are reliable for measuring changes in [Ca
2+]
i or [H
+]
i caused by acetate-induced cytosolic acidification
[20][21][20,21].
3. Gating by Heptanol in the Presence and Absence of Caffeine or Theophylline
Lateral giant axons have a membrane potential that ranges from −80 to −95 mV (Figure 2A and Figure 3A) and are electrically coupled at the septum with an initial Rj of 150 ± 53.7 kΩ (mean ± SD; n = 28). Superfusion of the axons with 2.8–5.6 mM heptanol results in a small depolarization (Figure 2A and Figure 3A) and increases Rj by 191.3 ± 83% (mean ± SD; n = 28) of control values (Figure 2B and Figure 3B). Note that the peaks of depolarization and Rj correspond well (Figure 2A,B). Recovery of both Rj and membrane potential (MP) are quicker than their onset (Figure 2A,B and Figure 3B).
Figure 2. Time course of changes in electrotonic potentials (
A) and Rj (
B) in septate axons treated first with heptanol and then with heptanol-caffeine (20 mM caffeine). The Rj recovery rate (
B) is faster than the onset rate with both heptanol and heptanol-caffeine. Note that the depolarization caused by heptanol ((
A), green arrow) is greater in the presence of caffeine ((
A), green and red arrows). Significantly, the peaks of depolarization correspond to the peaks of Rj (vertical red lines). Adapted from
[16].
Figure 3. Time course of changes in electrotonic potentials (
A) and Rj (
B) in axons uncoupled with heptanol in the presence and absence of 20 mM caffeine. Addition of caffeine to heptanol causes a rapid change in the amplitude of the electrotonic potentials (
A), indicative of rapid increase in Rj (
B). Rj increases with heptanol–caffeine 2–3 times as much as with heptanol alone ((
B), red arrow). The second heptanol treatment shows that the maximal rate of Rj rise with heptanol alone is 14 kΩ/min ((
B), green arrow), while with heptanol–caffeine (first treatment) the rate more than doubles (35 kΩ/min) ((
B), red and green arrows). Note that the membrane depolarization caused by heptanol ((
A), left green arrow) is increased by caffeine addition ((
A), red and green arrows). The maximum depolarization (
A) corresponds to the maximum Rj increase (
B). Adapted from
[16].
Addition of caffeine (10–20 mM) to heptanol solutions increases Rj maxima by 309.3 ± 265% (mean ± SD; n = 24) of controls treated with heptanol alone (Figure 2B and Figure 3B) and causes greater depolarization (Figure 2A and Figure 3A). Significantly, when caffeine is added to heptanol several minutes after the beginning of heptanol treatment, the rates of depolarization (Figure 3A) and Rj rise (Figure 3B) greatly increase. Indeed, a 7 min superfusion of heptanol–caffeine, following a 22 min superfusion of heptanol alone, virtually doubles the Rj rise induced by heptanol alone (Figure 3B). Following the first heptanol–caffeine application the Rj maxima with heptanol alone or heptanol–caffeine significantly decreased (Figure 4, blue and red arrows, respectively). This may suggest that the first heptanol–caffeine treatment reduced the calcium content of the endoplasmic reticulum (ER). Addition of 10 μM ryanodine partially reduces the Rj maxima reached with heptanol–caffeine (Figure 5), suggesting a partial inhibition of calcium release from the ER stores.
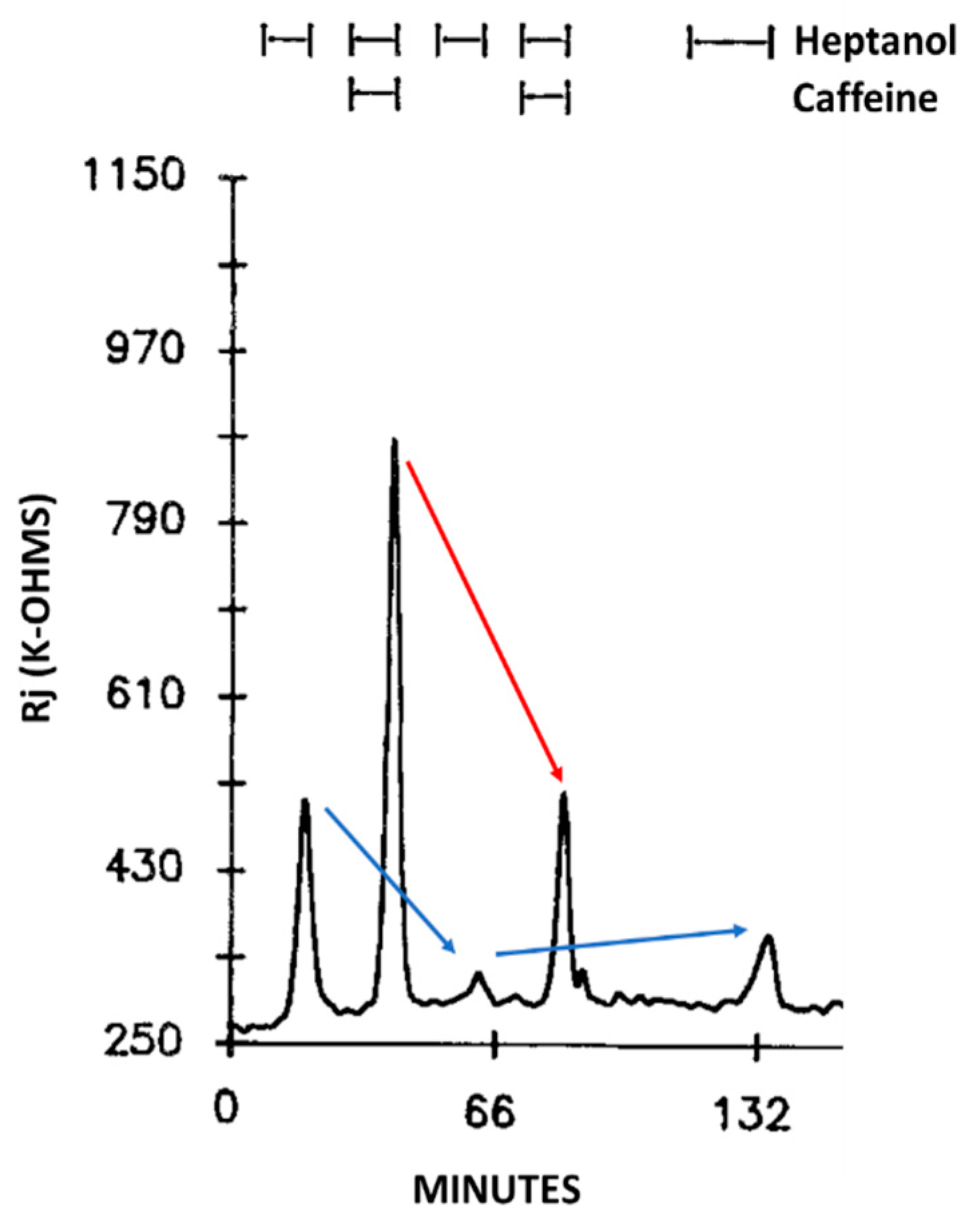
Figure 4. Time course of Rj changes in crayfish axons uncoupled by heptanol in the presence or absence of caffeine. Note the difference in Rj maxima between first and third uncoupling events (left blue arrow), in spite of the same duration of heptanol superfusion (8 min). Similarly, the Rj maxima decrease in the presence of heptanol-caffeine (compare second and forth uncoupling events; red arrow). The heptanol effect on Rj partially recovers (see 5th event, right blue arrow). The drop in Rj maxima in the 2nd and 4th event may indicate the first heptanol and heptanol-caffeine treatments lowered the Ca
2+ content of the Ca
2+ stores. Adapted from
[16].
Figure 5. Time course of changes in Rj in crayfish axons uncoupled by heptanol–caffeine (20 mM caffeine) in the presence and absence of ryanodine (10 μM). The caffeine-induced increase in heptanol-uncoupling efficiency is reversibly reduced by the addition of ryanodine. Adapted from
[16].
Addition of 10–20 mM theophylline to heptanol solutions also dramatically enhanced the heptanol effects on Rj (
Figure 6). Indeed, Rj maxima with heptanol–theophylline are 676 ± 386% (mean ± SD; n = 4) greater than those with heptanol alone. Neither different external [Ca
2+]
o, ranging from 7 to 27 mM, nor blockers of Ca
2+ entry, such as Cd
2+ (500 μM) or nisoldipine (10 μM), significantly change the heptanol-uncoupling efficiency
[16].
Figure 6. Time course of Rj in crayfish axons uncoupled by heptanol in the presence and absence of 20 mM theophylline. As with caffeine (
Figure 2,
Figure 3 and
Figure 4), theophylline dramatically increases Rj maxima with heptanol. Adapted from
[16].
The possibility that the effect of caffeine or theophylline is due to an increase in cyclic nucleotides was tested by exposing the axons to 3-isobutyl-1-methylxanthine (IBMX, a phosphodiesterase inhibitor), forskolin (an activator of adenylate cyclase) or diffusible cAMP and cGMP (CPT-cAMP and 8Br-cGMP)
[16]. Additions to heptanol of 1 mM IBMX (
Figure 7), a phosphodiesterase inhibitor 200 times more potent than caffeine
[22], 5 μM forskolin, 500 μM CPT-cAMP or 200 μM 8Br-cGMP do not significantly affect Rj maxima
[16]. The possible involvement of protein kinase C (PKC) was tested by superfusing the axons with heptanol solutions containing either 162 nM TPA (4β-phorbol-12β-myristate-13α-acetate) or 100 μM H7 (1-(5-isoquinoliny sulfonyl)-2-methylpiperazine); neither TPA (an activator of PKC) nor H7 (an inhibitor of PKC) significantly affected the magnitude of heptanol-induced uncoupling
[16].
Figure 7. Time course of Rj in crayfish axons uncoupled by heptanol in the presence and absence of 1 mM IBMX. IBMX (an inhibitor of phosphodiesterases and adenosine receptors) does not affect heptanol-induced Rj rise. Adapted from
[16].
In view of the fact that caffeine is also a powerful inhibitor of adenosine receptors, the effect of adenosine on Rj was tested both in the presence and absence of heptanol. Superfusion of 1.3–5 mM adenosine, added to either SES or heptanol solutions, does not significantly change either control Rj values or Rj maxima with heptanol, indicating that adenosine receptors do not participate in the effect of heptanol and caffeine on gap junction channel conductance
[16]. Lack of an involvement of adenosine receptor inhibition is also provided by the absence of an effect of IBMX on heptanol-induced Rj rise (
Figure 7)
[16]; indeed, IBMX, as caffeine and theophylline, is also an inhibitor of adenosine receptors
[23][24][23,24].
Curiously, the K
+ channel blocker 4-aminopyridine (4-AP) strongly inhibits the heptanol-induced uncoupling. With heptanol solutions containing 5 mM 4-AP, the Rj maxima are 26.2 ± 20% (mean ± SD; n = 12) lower than those with heptanol alone (
Figure 8A). In contrast, addition of 4-AP (5 mM) to acetate solutions does not alter their uncoupling effects (
Figure 8B). No effect on Rj was seen with 4-AP alone, with the only change being a 3–4 mV depolarization
[16], probably caused by the inhibition of K
+ channels.
Figure 8. Time course of Rj in crayfish axons uncoupled by heptanol (
A) or acetate (
B) in the presence or absence of 5 mM 4-aminopyridine (4-AP). 4-AP dramatically reduce the Rj maximum with heptanol (
A). In contrast, addition of 4-AP to acetate solutions (Ac) does not affect the acetate-uncoupling efficiency (
B). Adapted from
[16].