The detection of harmful gases and vapors is of great importance in relation to national defense
[1], monitoring environmental pollution
[2,3,4][2][3][4] and industrial emissions
[5[5][6],
6], and medical diagnosis
[7,8][7][8]. Especially, with the coming of the Internet of Things (IoT) era, the development of high-performance portable and wearable gas sensors able to work at room temperature has attracted great research interest. Single-wall carbon nanotubes (SWCNTs) composed of a single layer of sp
2-hybridized covalently-bonded carbon atoms have a unique one-dimensional tubular structure, high specific surface area, and excellent mechanical, electrical, thermal, and chemical properties. They are therefore considered an ideal candidate for the fabrication of high-performance gas sensors. Traditional gas sensors are usually assembled using metal oxide semiconductors, which work well in a high temperature range of 150~400 °C but this may decrease their sensing stability and lifetime
[9] and bring risks of combustion and explosion and high power consumption, which are undesirable for the next-generation portable and wearable gas sensors
[10]. In contrast, CNTs have a high sensitivity for target analytes at room temperature
[11], due to their enhanced adsorption rates of gases and vapors originating from their high surface area
[12]. Since Dai and co-workers
[13] first reported a chemical sensor fabricated using an individual CNT in 2000, notable progress has been made in the development of CNT-based gas sensors.
2.1. SWCNT Structure
The construction of an SWCNT can be conceptualized by rolling a perfect graphene sheet into a cylinder along the chiral vector
[9] [9]. Three types of SWCNT can be formed according to roll-up vectors (
n,
m). The (
n,0) structure is called “zigzag” and the structure where
n =
m (
n,
n) is called “armchair”. The third, where
n >
m > 0, is called “chiral”. The chirality determines the electrical, mechanical, optical, and other properties of SWCNTs. For example, an SWCNT can be either semiconducting (s-) or metallic (m-) depending on its chirality. Metallic and semi-metallic SWCNTs have roll-up vectors such that
n − m=3q (where
q is an integer) and semiconducting CNTs have
n − m=3q ± 1 . The distinction between semiconducting and metallic SWCNTs is important in the operation of nanotube-based field effect transistor (NTFET) devices
[10]. The strong covalent carbon-carbon bonds make SWCNT a superb structural material with an ultrahigh stiffness (up to 1 TPa) and tensile strength (experimentally approaching 80 GPa
[11]) in the direction of the tube axis. Meanwhile, the sp
2 hybridization gives it fascinating electrical properties that depend on the diameter and helicity
[12]. This combination leads to extraordinary mobility
[13] and excellent quantum ballistic transport
[14]. In addition, the large surface-to-volume ratio of SWCNTs, and their porous structure formed by interconnected tubes (or tube bundles) means that the carbon atoms are exposed to the environment and can be functionalized with abundant
[15] and effective binding sites for gas molecules
[16]. One of the most attractive features of SWCNT-based gas sensors is their ability to form flexible sensors for various gases
[17,18,19][17][18][19], as well as working at room temperature with a low power consumption
[20].
2.2. Sensing Principle
According to the International Union of Pure and Applied Chemistry, a chemical sensor is defined as a device that transforms chemical information, ranging from the concentration of a specific sample component to total composition analysis, into an analytically useful signal
[21]. Such devices are logically made up of two main components: the sensing material (or receptor) and the transducer (
Figure 21 in Molecules-10.3390/molecules27175381). Pure SWCNTs alone act as both the sensing material and the transducer, directly recognizing gases or vapors such as NO
2, NH
3, benzene and benzene derivatives with high affinity and transducing them into measurable signals. In addition, SWCNTs are a superb medium for functionalization that can detect insensitive gases towards pristine CNTs. SWCNT-based gas sensors can be classified according to the type of signal they produce, either electrical, optical
[22[22][23][24],
23,24], capacitive
[25] and acoustic
[26]. Among these, sensors that produce electrical signals are preferred due to their simplicity, portability, compatibility with standard electronics, and ability to be continuously monitored
[27].
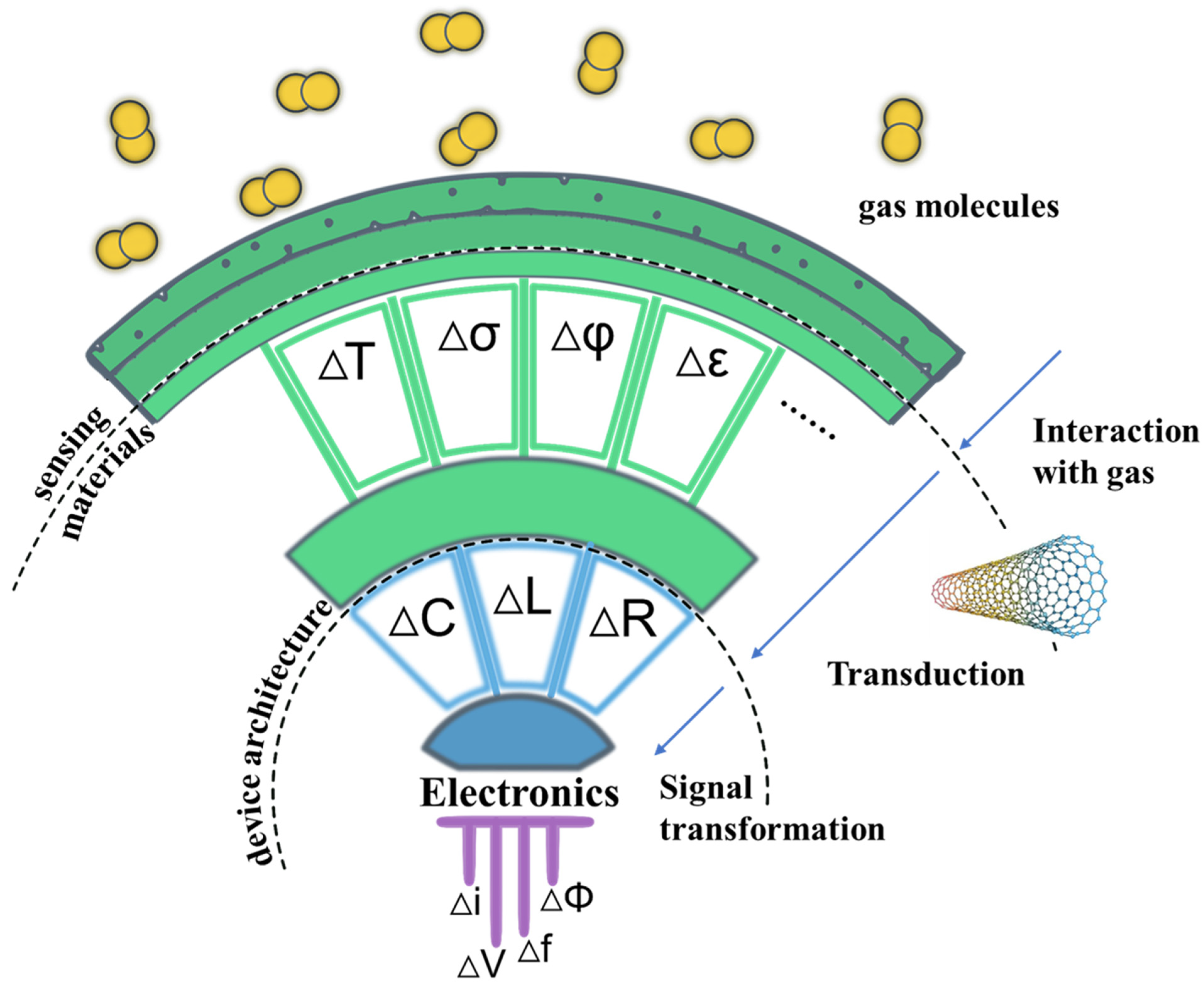
2.3. Important Figure of Merit of Gas Sensors
Figure 1. Logical structure of a gas sensor. Adapted with permission from American Chemical Society https://doi.org/10.1021/acs.chemrev.6b00361 (accessed on 4 August 2022) [28]. Analytes interact with the sensing material (CNTs or functional active sites on CNTs) changing some of its physical properties (e.g., temperature, ΔT; conductivity, Δσ; work function, ΔΦ; and permittivity, Δε). Transduction converts one of these physical quantities into a change in an electrical parameter (capacitance, inductance, and resistance are mentioned). Finally, the circuit connected to the sensor gives rise to a signal, usually a current or voltage change that can be measured.
2.3. Important Figure of Merit of Gas Sensors
An ideal gas sensor needs to have the following features: (i) high sensitivity to low gas concentrations, (ii) rapid response, (iii) reversible operating ability, (iv) good selectivity to different gases of interest, (v) low-manufacturing cost, (vi) stable operation over many cycles of use, and (vii) low power consumption during operation
[29].
Sensitivity is defined as the ability to discriminate small differences in the concentration of the analyte gas, and it can be calculated by the relative changes in the signal measured by the sensors, including resistance, current, conductance, capacitance, and power gain, depending on the type of sensor.
The response and recovery times are important factors when evaluating the performance of a gas sensor. The response time is defined as the time for the sensor to reach 90% of its steady state or maximum value on exposure to a given concentration of the analyte, while the recovery time is the time taken to recover 90% of its peak value
[32][30]. The response time is strongly dependent on the device structure, recognition components, and analytical techniques used to generate the signal
[30][31]. A fast response time is desired for the real-time and continuous detection of gases and their monitoring
[33,34][32][33].
LOD is the lowest concentration of target gas which can be reliably distinguished with a specified precision and reproducibility (typically with a 99% confidence level)
[27]. The LOD of a sensor can be influenced by receptor–analyte interactions, surface area, functionalization, and signal amplification
[37][34], and is closely tied to high sensitivity. The higher the affinity between target gas and SWCNTs (or functionalized SWCNTs), the lower the LOD, the faster the gas sensing response, and the harder the recovery of the sensors.
Drift is the slow, non-random change of signal with time while the concentration of the measured analyte remains constant. Although drift can be addressed either by in-device recalibration or algorithms during data processing and/or workup, many applications cannot sustain intensive computational solutions to sensor drift
[37][34]. Drift is undesirable for practical sensors and remains a challenge to be solved for CNT-based sensors.
Selectivity is the ability of a sensor to identify the target gas present in a sample containing several other interfering chemicals
[21]. Although the selectivity of pristine SWCNTs is usually poor due to their robust and stable C-C covalent bonding, some CNT-based sensors have demonstrated a satisfactory selectivity with the help of functionalization
[17,27,42,43][17][27][35][36].
A field-effect transistor (FET) using SWCNT(s) as the active channel is a versatile sensor platform. The simplest FET consists of two electrodes (the source and the drain), connected by a semiconductor as the channel, and a gate electrode located typically at the back of an insulating gate oxide substrate that applies a gate voltage to modulate the channel current, which provides additional means to control the current response in the channel material when it interacts with a target gas. The amplification effect of FETs makes them easy to be used as gas sensors that can detect weak signals caused by trace amounts of gases, and are expected to blaze a novel trail in the field of trace gas detection
[36][37]. FET-structure SWCNT-based sensors commonly have a better sensitivity
[30][31] and provide more data for sensing analysis than a resistive sensor.
The most common structure is a resistive sensor, where only two electrodes are used. In the absence of the gate electrode, this structure is simpler than transistors, leading to a low cost, making it available for widespread use. The sensors show changes in conductivity when exposed to target gases but are not suitable for further investigation of working mechanisms. Sometimes, although a sensor is fabricated with the configuration of FET, it has resistive behavior
[44][38] due to the metal decoration on SWCNTs.
2.4. The Origin of the Sensing Response
The frontier orbitals of SWCNTs described using the band structure are better at predicting or describing the sensing mechanisms
[32][30], according to solid-state physics. The responses of the SWCNT-based sensors are attributed to effects resulting from (a) contact between the tubes and the electrodes (Schottky barrier modulations); (b) the sidewall or the length of the tubes (intra-SWCNT); or (c) contact points between nanotubes (inter-SWCNT). To some extent, all these sites can be regarded as effective sites. For sensor devices consisting of a network of SWCNTs, responses at the interfaces between nanotubes may be significant to the electronic properties of the overall network, because the distance between tubes might be changed by the gas absorption
[45][39]. The active sites that dominate the response may differ with the analyte, the type of SWCNT, and the device structure
[46][40].
Electrical measurements on resistive sensors cannot provide sufficient information to elucidate their gas sensing behaviors, but I–V testing of SWCNT FETs can distinguish the different sensing mechanisms. When a metal contacts SWCNTs, a potential barrier arises due to the difference in their work functions, as a result of which the junction may exhibit rectifying characteristics, which is called a Schottky barrier. Under a constant bias voltage, the conductance of semiconducting SWCNTs can be changed by changing the gate voltage (V
G), which modifies the Schottky barrier and therefore the probability of a hole (h+) traveling from the metal contact into the CNT valence band
[10].
3. Approaches to and Progress in Improving the Sensing Performance
3.1. Pure SWCNT-Based Sensors Produced by Tuning the Structure
A pure SWCNT-based sensor is able to detect target gases at trace concentrations, and the sensing performance varies with the source of the SWCNTs
[56][41] even with same the pre-treatment. The quantity and morphology of SWCNTs play an important role in determining the gas sensing performance
[32[30][42],
57], with parameters such as tube density (from individual to networks), an isolated or bundled state, type and concentration of defects, and amounts of metallic or semiconducting tubes need to be taken into consideration.
3.2. Functionalization of SWCNTs
As described above, much progress has been made in SWCNT-based gas sensors by controlling the network structure, defects, and conductivity type of SWCNTs. However, the inertness of sp
2 carbon makes a pure SWCNT-based sensor have a low sensitivity for analytes such as H
2, CH
4, and CO
2 [6,93][6][43]. Furthermore, it is difficult to selectively detect a target gas in a gas mixture using pure SWCNT sensors. In order to improve the sensing performance, receptors that selectively recognize, interact or react with the target gas are commonly anchored on the surface or ends of the SWCNTs. Various methods have been proposed to modify SWCNTs, which can be classified as covalent and noncovalent functionalization
[94][44]. If the receptor reacts to form a covalent bond with the SWCNTs it’s called covalent functionalization. Covalent functionalization is strong and stable but lowers the intrinsic electronic properties of SWCNTs
[32][30]. Therefore, the degree of functionalization must be carefully controlled to achieve an optimum result. Noncovalent functionalization mainly involves the absorption of molecules containing receptors which are attractive because they produce less perturbation to the intrinsic properties of SWCNTs. The drawback of noncovalent functionalization is that it is not stable, which limits the working conditions of the sensor.
4. Conclusions
SWCNTs are one of the most promising materials for fabricating flexible gas sensors due to their unique geometries and extraordinary intrinsic properties, as well as their ability to be tailored to detect target gas molecules. SWCNT-based gas sensors have shown an excellent sensing performance including a fast response time and an extremely low LOD at room temperature. Considering the cost and efficiency, SWCNT networks comprised of isolated s-SWCNTs with an appropriate degree of functionalization could be a promising candidate sensing material. Combining the design of sensor structure and configuration with SWCNT flexibility and transparency, SWCNT-based gas sensors may have great promise for use in the IoT, wearable devices and aerospace applications.