1. Introduction
Sewage-water treatment plants (SWTPs) comprehend sewage-water management and the waste produced by the treatment process. The treatment steps within a SWTP are usually categorized as the primary, secondary, and tertiary levels of treatment. At the primary level, physical barriers remove coarse solid material, and the remaining solids are removed in sedimentation units. The secondary level removes soluble organics and suspended organics, while the tertiary level removes the remaining inorganics and other diluted organics as a finishing step
[1]. However, sewage sludge is generated in large volumes during sewage-water treatment, and its disposal represents up to 50% of the current operating costs of a SWTP
[2].
Aerobic and anaerobic digestion are typical strategies to reduce the sewage-sludge volume. However, the latter is preferable due to its concomitant biogas production
[3]. Anaerobic digestion (AD) converts the organic matter—expressed as chemical oxygen demand
COD (kg
COD/m
3) or as biochemical oxygen demand
BOD (kg
BOD/m
3)—of sewage sludge into biogas with 40–65% mol CH
4 [4], reducing the sludge disposal volume
[5]. Thus, with the biogas from AD, modern SWTPs are not limited to reusable water production but can also become self-sufficient in energy and export the surplus of produced power to the grid if a biogas-fired power plant operates jointly
[6]. Given the current climate change concerns, it must be noted that there is a stringent recommendation that the biogas from AD be collected and burnt to avoid much more harmful greenhouse gas (GHG) CH
4 emissions. Hence, it is natural that designers now plan SWTPs to be fully integrated with biogas-fired power plants
[7][8][9].
2. Power Generation in Sewage-Water Treatment Plants
A challenge in SWTPs is to feasibly reconcile sludge management and energy production. Combined heat and power (CHP) technologies that use biogas from AD are approaches devised to produce power to achieve energy self-sufficiency while reducing sludge disposal. Silvestre et al. (2015)
[10] analyzed SWTPs from an energy point of view and concluded that 67% of the initial energy content in the raw sewage water is transferred to sewage sludge. Then, at least 34% of sewage-water energy content can be recovered into biogas after AD. Udaeta et al. (2019)
[11] evaluated the electricity generation potential from a SWTP in São Paulo (Brazil) to supply domestic demand and export biogas or electricity surpluses. In their results, the methane produced by this SWTP represented 4% of São Paulo gas demand and could generate 762.8 GWh/y, or 5% of São Paulo electricity demand. Mensah et al. (2021)
[12] evaluated a SWTP with biogas production and electricity generation in Benin, West Africa. Agreeing with the conclusion of Udaeta et al. (2019)
[11], the unexplored potential of biogas can supply 2% of the electricity imported by Benin. In addition, the avoided cost of public health was estimated at USD 40 million over a 15-year period.
An evaluation of an energy self-sufficient SWTP was carried out by Schaubroeck et al. (2015)
[13], showing that the AD of sewage sludge enriched with kitchen waste and fats increased the methane content in the biogas and provided an additional 0.200 kWh/m
3 of usable power. Two examples of the energy self-sufficiency of municipal SWTPs with CHP units were presented by Nowak et al. (2011)
[14], wherein the biogas produced was used in CHP units reaching 40% thermodynamic yield, accompanied by 96%
COD removal with 80% nitrogen removal. However, the CHP system used by Nowak et al. (2011)
[14] presented only 7% of electricity surplus, because the major fraction of electricity was consumed for air compression, aeration, and stirring of the MAD tanks. Chen et al. (2016)
[15] evaluated four possible cases to enhance the CHP scheme with an absorption chiller in a system with 1409 kW of power output. The thermodynamic yield significantly improved from 20.8% to 38.3% in the best case, with 2–3 years of payback time. Similarly, Sadhukhan (2014)
[16] presented a feasible application of sewage-sludge AD, wherein the biogas was utilized in six different CHP technologies up to 500 kW (
Figure 1c): (i) biogas engine; (ii) ignition biogas engine; (iii) micro-gas turbines (Micro-GT); (iv) proton exchange membrane fuel cell; (v) solid oxide fuel cell (SOFC); and (vi) SOFC gas turbine (SOFC-GT). The Micro-GT presented the most cost-effective results, accompanied by a reduction in GHG emissions of 0.0982 kg
CO2eq/MJ
Electricity.
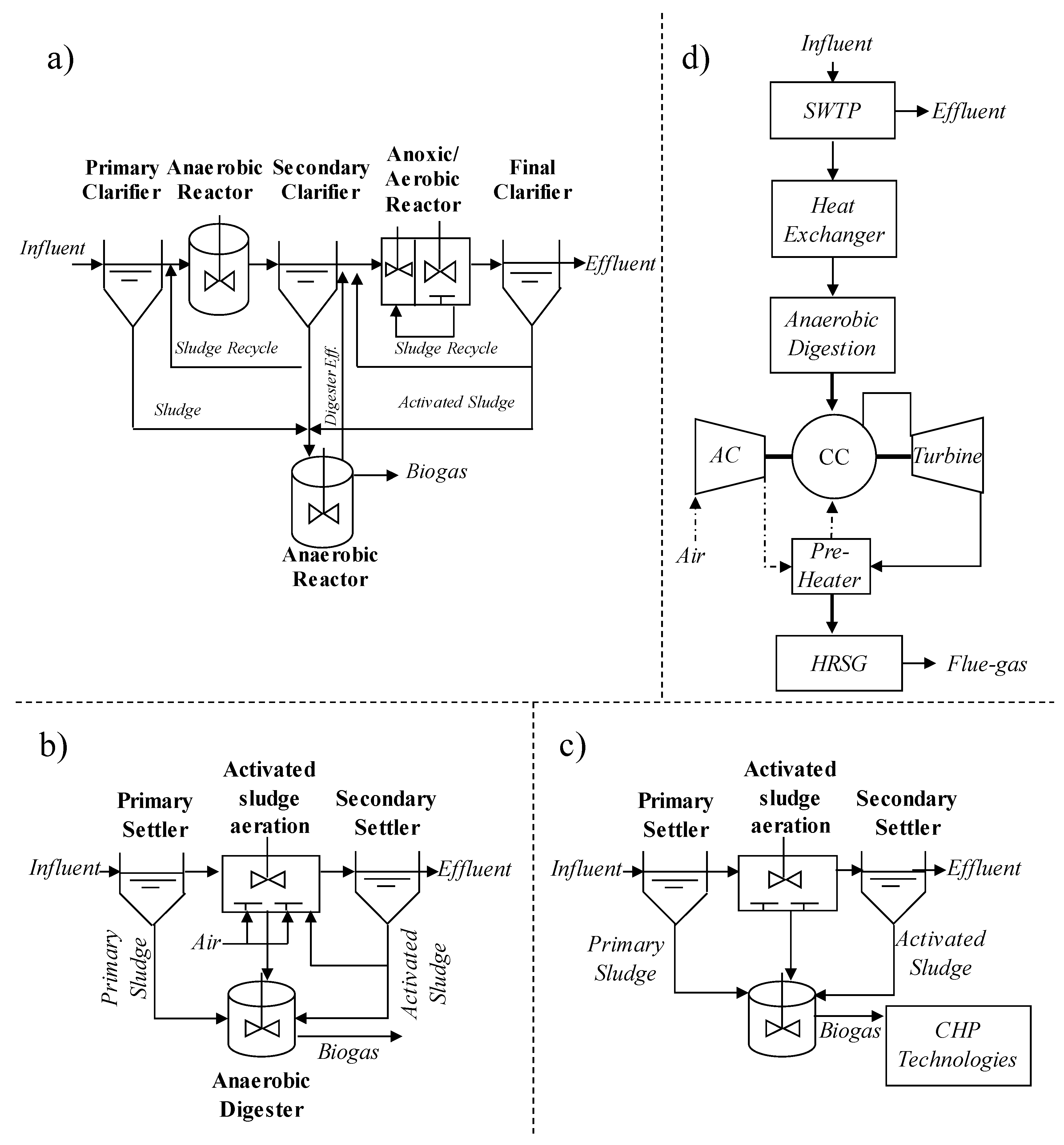
Figure 1. (
a) SWTP with sewage-sludge AD
[17]; (
b) primary and secondary treatments with sludge AD for biogas and power production
[18]; (
c) SWTP with sewage-sludge AD and biogas applications in CHP technologies
[19][18]; (
d) SWTP with AD and GT burning biogas
[19] [20] (AC: air compressor; CC: combustion chamber; CHP: combined heat and power; HRSG: Heat Recovery Steam Generator).
2.1. Biogas Gas Turbine Cycles and Biogas Combined Cycles
GTs and Micro-GTs comprehend an air compressor, combustion chamber, turbine expander, and electric generator. GTs are viable in a SWTP with a high biogas supply. A GT feature is the production of exhaust gases at a high volumetric flowrate and temperature. Moreover, a Micro-GT is essentially the same equipment, but with a small size for a smaller gas flowrate. Micro-GTs have typical power below 1000 kW, while GTs are sized above 1 MW
[17].
A recent and viable solution for electricity generation in SWTPs is applying a GT as a single Biogas GT Cycle or as a Biogas GT Cycle combined with a steam Rankine cycle (Biogas Combined Cycle). In this context, Basrawi et al. (2012)
[18] evaluated the isothermal AD of sewage sludge in which the produced biogas feeds the Micro-GT. The 30 kW, 65 kW, and 200 kW power units produced electricity at 40% of thermodynamic yield. In another work, Basrawi et al. (2017)
[1920] analyzed the optimum size of Micro-GT cogeneration for a SWTP in terms of its economic performance. They showed that the best net present value (NPV) is obtained when different sizes of Micro-GTs are combined to meet the available feed flowrate, approximately equal to the biogas production. Chang et al. (2019)
[2019] also presented a case study using a 30 kW Micro-GT fed with biogas from the AD of sewage sludge. The biogas production of 4424 m³/d in Chang et al. (2019)
[2019] is very similar to the 4321 m³/d reported by Basrawi et al. (2012)
[18], with a slight difference in biogas composition (CH
4/CO
2): 60/40 in Chang et al. (2019) and 56/25 in Basrawi et al. (2012)
[18]. In addition, Chang et al. (2019) reported that the SWTP consumed 25.82% of the biogas for heating, and the remaining was used for electricity generation at 172,003 kWh/y with a thermodynamic yield of 0.234 kWh
Electric/kWh and electricity generation efficiency of 1.09 kWh
Electric/m³
Biogas.
Another recent research trend in the context of SWTP power production is to integrate AD modeling with simulated Biogas GT Cycles or AD modeling with simulated Biogas Combined Cycles—i.e., GT Cycles integrated with steam Rankine cycles via Heat Recovery Steam Generators (HRSG)—for power production, with or without carbon capture and storage (CCS). Lee et al. (2017)
[21] presented a SWTP-CHP-AD system (
Figure 1d) where the mesophilic AD was simulated via Benchmark Simulation Model No. 2 (BSM2). Although Lee et al. (2017)
[21] did not present the AD inlet composition (V
Reactor = 3400 m³, SRT = 19 d), a production of 2463.2 kg
biogas/d was fed to a Micro-GT, which was optimized to manipulate the temperature differences in the heat exchangers and the GT compression ratio. The power generated in the optimized system supplied 47% of the SWTP’s power demand and 100% of its heat demand. Differently from Lee et al. (2017)
[21], Poblete et al. (2022)
[22] designed and simulated a large-scale—GHG negative emission—SWTP in
Figure 2, with optimized bioenergy production via Biogas Combined Cycle, integrating GT Cycles via HRSG to Rankine cycle running steam turbines, wherein the GTs were fed with the biogas production modeled via ADM-1 simulation. In this research, two GTs fired 205,600 m³/d of biogas, providing 14044 kW of power and an additional 8227 kW from the Rankine cycle, assuming no CCS in the flue gas. In the negative-emission case, Poblete et al. (2022)
[22] showed that the Rankine cycle produced only 1928 kW due to the energy penalty of post-combustion CCS treating flue gas, but the captured CO
2 can generate auxiliary revenues if exported as a dense enhanced oil recovery agent (
Figure 2). Despite the impressive reduction in power brought by CCS, this research demonstrated that a SWTP operating as a Bioenergy with CCS (BECCS) system still has the potential to reach economic feasibility while promoting negative emissions, which is helpful in the climate-change scenario. Movahed and Avami (2020)
[23] also employed ADM-1 to calculate the biogas produced from the primary and secondary sewage-sludge processing. However, differently from Poblete et al. (2022)
[22], this research did not consider treating the flue gas for CCS and also did not consider the more efficient Combined Cycles, i.e., the biogas was fired in eight Micro-GTs with 100 kW power output instead of using large GTs coupled with HRSG and Rankine cycle.
Figure 2. Conceptual BECCS SWTP from Poblete et al. (2022) [22] (Lines: Solid = material streams, Dashed = electricity).
2.2. Internal Combustion Engines
Internal combustion engines firing biogas are a long-established and reliable technology for co-generation systems (Mustafi et al., 2006)
[24]. Henham and Makkar (1998)
[25] experimentally evaluated the application of biogas in electricity generation systems with dual-fuel internal combustion engines. The experiments used a wide range of CH
4/CO
2 ratios to emulate biogas from different sources, including SWTPs. The authors concluded that after modifications in the ignition system, it is possible to use up to 60% biogas as fuel with low efficiency losses. Gómez et al. (2010)
[26] estimated the cost of electricity generation from sewage-sludge AD using internal combustion engines in Spain. The sewage-sludge potential was estimated at 142 MWh
Electric/y with a minimum electricity cost from sewage sludge at 0.11 €/kWh, which can be reduced exploiting an AD economy of scale.
2.3. Integrated CHP Systems
SWTPs can use CHP systems to enhance the energy recovery from sewage sludge through biogas utilization. In this regard, integrated CHP systems have been developed to increase energy recovery. Di Fraia et al. (2018)
[27] proposed an integrated system for drying sewage sludge, with electricity production powered by the biogas from sewage-sludge AD coupled with solar energy collectors. The electricity produced by CHP was used to supply the SWTP’s demand. The thermal energy from the CHP unit, combined with solar energy, was employed to run the AD and thermal drying of the residual sludge. The solar collector has a thermal efficiency of 56%, and the CHP unit has 44% efficiency. However, they achieved a small percentage (~14%) of the energy recovery obtained by González-Arias et al. (2020)
[28] via the integration of AD with pyrolysis, which can double electricity generation. González-Arias et al. (2020)
[28] evaluated an integrated AD process with the pyrolysis of sewage sludge from AD, in which the sewage sludge was blended with fats and was submitted to mesophilic AD (
T = 35 °C, HRT = 40 d). Results demonstrated that adding 3%
w/
v of fat to the sludge feed promoted a 25% increase in the generated electricity from biogas in the CHP unit. Moreover, increasing the fat ratio to 15%
w/
v allowed an increase of 2.4 times the electricity generation while fulfilling all heat needs for sludge drying. Confirming the approach of González-Arias et al. (2020)
[28] as one of the most sustainable options, Mills et al. (2014)
[29] showed the life-cycle assessment results for several configurations with CHP. The best economic and environmental performances were achieved for thermal hydrolysis AD coupled with CHP, followed by drying the digested sludge for solid fuel production and pyrolysis.
2.3.1. Solid Oxide Fuel Cell
The utilization of biogas in SOFCs is a promising way to achieve an energy self-sufficient SWTP and has been experimentally investigated at lab-scale. Giarola et al. (2018)
[30] compared the performance of a SOFC system integrated with a SWTP with conventional alternatives and concluded that SOFCs may become cost-competitive in thermally optimized SWTPs. Mehr et al. (2018)
[31] modeled a large-scale SOFC-CHP-SWTP, in which the biogas from sewage-sludge AD was reformed to H
2 and CO. The authors concluded that a 27% savings of energy cost could be achieved. Wang et al. (2021)
[32] analyzed the performance and operation parameters of biogas-fed SOFC-CHP-SWTP systems. The results showed that increasing the CH
4 fraction in biogas leads to greater electrical efficiency, showing that SOFCs for biogas applications are competitive with other co-generation technologies.
2.3.2. Photovoltaic Systems
Gretzschel et al. (2020)
[33] conducted a SWTP feasibility study that implemented water electrolysis for H
2 and O
2 productions driven by photovoltaic sources and CHP-SWTP units to provide the required electricity. The obtained H
2 fed the local gas grid, and the O
2 was used for micro-pollutant removal via ozonization of the sewage water. The photovoltaic power generation was 227 MWh/y, while the CHP unit produced 6173 MWh
Electric/y at 38.5% thermodynamic yield. In an attempt to upgrade the biogas heating value, Su et al. (2017)
[34] considered solar energy to reform biogas and improve the energy performance of CHP. The obtained synthesis gas was efficiently converted in the CHP to electric power and heat for the digester, saving 142.757 Nm³/y of biogas. In contrast to Gretzschel et al. (2020)
[33] and Su et al. (2017)
[34], Jacob et al. (2020)
[35] did not use photovoltaic electricity to produce or modify biogas composition. They proposed photovoltaic technologies to supply the electrical and thermal demand of a SWTP, enabling them to sell the produced biogas as biomethane. The results showed significant economic and environmental gains over the conventional CHP, entailing internal return rates of 5–12.7%.
3. Power Generation in Sewage-Water Treatment with Carbon Capture and Storage
The growing climate change concerns motivate the minimization of GHG emissions and fossil energy consumption by SWTPs. However, the enormous volume of generated solid waste underlines the necessity of a sustainable way to handle it (Wei et al., 2020)
[36]. Fighir et al. (2019)
[37] remark that the massive GHG emissions of large-scale SWTPs come from firing biogas for heat production and CH
4 emissions from AD, which has a global warming potential (GWP) 21 times greater than CO
2. Bani Shahabadi et al. (2010)
[38] studied a typical SWTP integrated with a Biogas Combined Cycle (BGCC) power plant fed with 1000 m³/d (~2.0 kg
BOD/m
3) of sewage water. They estimated a carbon credit of 34 kg
CO2eq/d due to methane elimination in the BGCC instead of using conventional natural gas.
Life-cycle assessments of power-producing SWTPs are used to analyze the GWP and GHG emission of sewage-sludge treatment technologies. Buonocore et al. (2018)
[39] results showed that AD was essential to achieve energy self-sufficient SWTPs, and the use of treated sewage water for irrigation as a final disposal reduces eutrophication of water bodies by 60%. Borzooei et al. (2020)
[40] analyzed the carbon footprint and reported a 40% reduction in GHG emissions, considering the upgrading of biogas to biomethane, which is sent to the natural gas distribution grid. Keller and Hartley (2003)
[41] concluded that GHG emissions from SWTPs are mainly driven by the selection of aerobic or anaerobic treatment for sludge management. In their study, the GHG emission of a SWTP combining AD+MAD resulted in a reduction of 1.4 kg
CO2/kg
CODRemoved compared to a fully MAD treatment. Niu et al. (2013)
[42] approached sludge management and demonstrated that biogas utilization is crucial to reduce up to 24% of the total carbon footprint of SWTPs. Moreover, AD is responsible for contributing about 90% of GHG emissions when heat provided by the CHP units is insufficient to keep the temperature at 38 °C. In accordance with Buonocore et al. (2018)
[39], Niu et al. (2013)
[42], and Keller and Hartley (2003)
[41], Münster and Lund (2009)
[43] presented an energy analysis of different SWTP technologies that utilized organic waste for heat power production. They concluded that the best solution for minimizing GHG emissions is using organic waste to produce biogas and, consequently, CHP power production.
Chernicharo et al. (2017)
[44] evaluated the management of GHG emissions from UASB-based SWTPs, considering the carbon footprint as the main deciding factor. The CHP unit was feasible and avoided 201.3 t
CO2eq/y, with exhaust gases used for the dehydration of thermal sludge reducing sludge volume and transportation fuel consumption. Maktabifard et al. (2019)
[45] achieved a reduction of ~30% carbon footprint by applying energy recovery from CHP, a reduction slightly higher than that presented by Niu et al. (2013)
[42]. Maktabifard et al. (2019)
[45] also found that 8% of the carbon footprint could be reduced by substituting synthetic fertilizers with AD digestate. Campello et al. (2020)
[46] evaluated the energy potential and the economic viability of SWTPs in Brazil. Their results indicated that biogas recovery systems are feasible in most cities, reaching a
payback time of
8 years. Their findings also included an estimated potential electricity generation of 47,140 MWh/y and a potential reduction in GHG emissions close to 325,800 t
CO2eq/y.
The use of negative-emission energy-producing technologies—or BECCS systems—is a recently proposed strategy to reduce the carbon footprint of large-scale power-producing processes. BECCS processes are large-scale bioenergy production systems coupled with permanent CO
2 drainage from the carbon cycle, mitigating (or reverting) climate changes through systematic long-term negative emissions
[47]. Pour et al. (2018)
[48] presented the BECCS potential of municipal solid waste management via the integration of incineration and landfill processing with CCS. Ziółkowski et al. (2021)
[49] modeled a BECCS power plant based on the gasification of sewage sludge. However, there are still few works in the literature that address BECCS configurations of full SWTPs handling water recovery, waste management, energy production, and carbon abatement simultaneously
[50]. Poblete et al. (2022)
[22] pioneered a BECCS large-scale SWTP case study (
Figure 2) that treated 10 kgCOD/m³ sewage water in AD + MAD reactors, producing 60% mol CH
4 biogas, which was used to produce bioenergy to supply electricity to the CCS plant and export the surplus to the grid. The main conclusion from Poblete et al. (2022)
[22] was to demonstrate the techno-economic feasibility of a BECCS-SWTP accompanied by 9.95 t
CO2/h of negative CO
2 emissions, i.e., the large-scale BECCS-SWTP in
Figure 2 can accomplish six simultaneous beneficial targets: (i) it recovers a great flowrate of reusable water from sewage water; (ii) it is self-sufficient for heating (via low-pressure steam) and electricity and even exports some; (iii) it is economically feasible and self-sustainable; (iv) it does not emit carbon to the atmosphere, thanks to its implementation of CCS treatment of flue gas; (v) it generates extra revenues by exporting the captured dense CO
2 for enhanced oil recovery (i.e., CO
2 is traded for oil); and (vi) it even combats climate change by draining CO
2 from the atmosphere, as sewage solids partially come from vegetable sources.
Expanding the application of the BECCS concept, Poblete et al. (2020)
[51] assessed the performance of a BECCS configuration of a small-scale rural AD plant that processed animal wastes with biogas production. In this case, biogas fed a Biogas Combined Cycle (BGCC) power plant with CCS. Energy storage is implemented via dynamic Compressed Air Storage (CAS) in order to store power production during nighttime periods of low power consumption. The research claimed the feasibility of BECCS-BGCC-CCS-CAS in a carbon taxation scenario with 1.03 tCO
2/h negative emission. Unlike Poblete et al. (2022)
[22] and Poblete et al. (2020)
[51], in which biogas production and waste management gave rise to a BECCS process, in the works of Pandey et al. (2016)
[52], Huang et al. (2016)
[53], and Lu et al. (2015)
[54], CCS is performed via microbial electrolytic carbon capture (MECC). Wastewater was used as an electrolyte for microbial electrolytic carbon sequestration and hydrogen production. Unlike AD, MECC can operate at low temperatures
(~4 °C), presenting low sludge production and offering potential treatment for a wide range of organic contaminants of wastewater. However, this type of technology is still immature, and the MECC costs are still barriers that limit its applications. Similar to MECC, microbial electro-synthesis (MES) differs in the cathode reactions and the production of bio-commodities from sewage water
[55]. In Batlle-Vilanova et al. (2019)
[56], the biogas produced in the AD of sewage sludge was upgraded by using MES, increasing the content of methane in the biogas and producing chlorine for the disinfection of treated sewage water.