2. Recent Trends
Conventionally used ferromagnetic materials exhibit some limitations, such as a low hardness, which hinders their application and has necessitated the development of better ferromagnetic materials
[6]. When ferromagnetism was unexpectedly observed in Ge, Mn, and Te, several studies were conducted on inorganic nonmetallic materials, and many ferromagnetic materials were successfully discovered. Traditionally, ferromagnetism was believed to be demonstrated by elements with partially filled d or f orbitals. In contrast, when researchers revealed that an element without partially filled d or f orbitals could also exhibit ferromagnetism, it opened up a completely new realm in the field of ferromagnetism. Reducing one of the dimensions limits the electronic transition, promoting column interactions and increasing the bandwidth ratio. This increase in material–column interactions and bandwidth ratio induces magnetism in these materials
[7]. Numerous materials with either partially filled f or d orbitals were both theoretically and experimentally analyzed. Nonmagnetic elements that show ferromagnetism in their oxide compounds or other forms are referred to as d
0 ferromagnetic substances, and the corresponding ferromagnetism is termed d
0 ferromagnetism. d
0 Ferromagnetism was discovered for the first time in HfO
2 when the O-rich surface of Hf with no magnetic ions in HfO
2 exhibited ferromagnetism
[8]. Thereafter, the ferromagnetic properties of oxides of other elements of the same period, including ZnO, Cu
2O, and TiO
2, were examined, and these oxides were found to demonstrate ferromagnetism; furthermore, numerous other nonmagnetic d-block elements, such as Sc, Cr, Mn, Zr, and Nb, were reported to exhibit ferromagnetism upon doping with some specific type of impurities
[9]. Similarly, oxides of f block elements, such as CeO
2 doped with Co and Mn, were investigated to further explore this
[10]. Moreover, the oxides of nonmagnetic elements without d or f orbitals, including Al
2O
3, In
2O
3, and CaO, demonstrated ferromagnetic properties, implying that these properties can be modified within elements
[11]. Subsequently, the non-oxide nonmagnetic compound BN, C structures, and rock salts were also reported to possess considerable ferromagnetism
[12]. Accordingly, it was concluded that modification of the internal environment of a nonmagnetic element or a ferromagnetic nonmetal by defects, such as valency complexes, vacancies, and impurities, arising from the integration of the element with another element, can induce magnetic properties
[13]. Thus, the phenomenon of ferromagnetism is universal, and is demonstrated not only by magnetic elements, but also their impurities and non-magnetic elements by doping with oxide or other inorganic elements
[14]. Furthermore, the drawbacks that limit the use of traditional magnetic elements have been overcome by mixing these elements with other compounds; for example, B
[6]. Many modern medical procedures, such as varicose treatment, hyperthermia, and endovenous thermal ablation require local heating of the human body. However, it is challenging to convert electrical power into heat flux and directly transfer this heat flux to the required area without harming the surrounding tissue. Heating catheters composed of biocompatible magnetic composites with low-frequency induction heating (LFIH) is an effective solution in this regard
[15]. Cu–Mn–Ga-based ferromagnetic shape memory single crystals were fabricated for the first time by annealing their cast polycrystalline alloys. Their functional properties have also been reported. The obtained results should be highly significant for the development of brittle ferromagnetic Cu–Mn–Ga alloys
[16]. Numerous studies have been performed on the advancement of ferromagnetism, which have resulted in the discovery of numerous ferromagnetic compounds.
2.1. Hexaborides of Alkaline-Earth Metals
Boride compounds are gaining considerable attention because of their mechanical properties and high conductivities. They are widely used to overcome the hardness limitations of magnetic elements
[6]. B, as an electron-deficient element, facilitates the trapping of electrons from other metal species by forming a strong B bond with these species. They produce either divalent-metal hexaborides or divalent hexaborides with alkaline-earth metals
[17]. The hexaborides of alkaline-earth metals, including SrB
6, BaB
6, and CaB
6, are extremely sensitive to stoichiometry because of their high impurity contents and inferior physical properties. When these compounds were doped with Th and La, the resulting compounds demonstrated weak ferromagnetism at high temperatures
[18]. Although these compounds lack d or f orbitals, their ferromagnetism has attracted significant research attention
[9]. Divalent hexaborides (MB
6, M = Sr, Ba, Ca) crystallize in CsCl-type cubic structures, and their physical properties are substantially similar to those of Group IIA elements, that is, alkaline-earth metals
[19]. The structure of a divalent hexaboride comprises a central metal ion surrounded by hexaboride ions (
Figure 2). In the CaB
6 prototype structure, a single metal atom is surrounded by eight octahedra of B atoms, each centered on the corner of the cube. There are five B atoms in an octahedron, with four adjacent atoms and one along one of its axes. Moreover, 24 B atoms are coordinated at the center of the atom; nevertheless, no valence bonds are formed between them
[20]. In contrast, the B atoms are covalently bonded to each other and are responsible for several physical and chemical properties of these compounds
[21]. A cubic B sublattice is inherently electron-deficient because the B atoms must distribute their three valence electrons over five bonds. This implies that a sublattice cannot exist, even with the donation of electrons from the metals. Hexaborides must contain a metal cation with a charge of at least +2 to be electronically stable
[22]. KB
6 with a low K content has been synthesized in controlled environments
[23]. Consequently, considering that the B sublattice forms a semi-rigid “cage,” alkaline-earth, rare-earth, and some actinide cations are the only candidates in this regard based on their sizes and valences.
Figure 2. Crystal structure of the hexaborides of alkaline-earth metals
[24].
2.2. Nonmagnetic Oxides
Numerous studies have been conducted on nonmagnetic oxides, and several other oxides of nonmetallic elements demonstrating ferromagnetism at room temperature have been discovered; nevertheless, there remains a knowledge gap regarding the origin of ferromagnetism in these compounds. Impurities and surface defects are the topic of controversies that are often discussed whenever the discussion arises
[25]. Ferromagnetism has been discovered in different oxides, for example, Mg, Zn, Ce, Hf, Ti, Sr, Sn, and Zr oxides, disproving the traditional concept of the requirement of d or f orbitals to exhibit ferromagnetism. Among them, oxides of Zn, Mg, Hf, and Ce are widely used and have extensive applications, whereas the remaining oxides exhibit weak ferromagnetism in the free state or after doping
[17].
2.3. C Nanostructures
Ferromagnetically active C systems have emerged as one of the most popular research topics in the field of ferromagnetism owing to their various advantages including low weight, high stability, low-cost production, and simple processing. Recent years have witnessed significant advancements using graphene (
Figure 3a), C nanotubes (
Figure 3b), fullerenes (
Figure 3c), and graphite (
Figure 3d)
[26]. As classic ferromagnetic substances lack d and f orbitals, ferromagnetism in C nanostructures originates from s and p electrons. These nanostructures demonstrate ferromagnetism at low temperatures, and the magnetism is due to the impurities present in these nanostructures
[27].
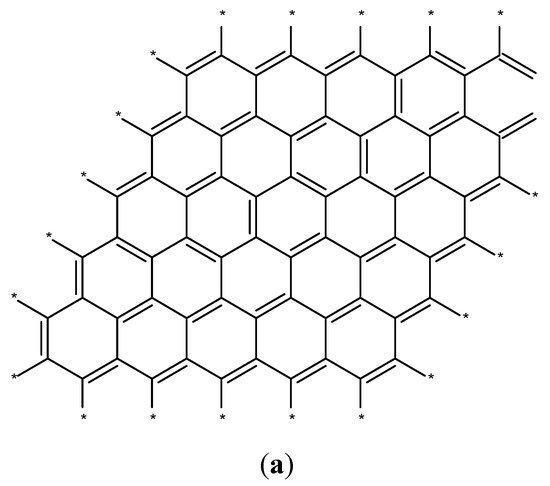
Figure 3.
Schematics of the structures of graphene (a
), C nanotubes (b
), fullerenes (c
), and graphite (d
) .
2.4. Magnetic Boride Compounds
B has the potential to form a wide range of compounds with different electrochemical, magnetic, and stoichiometric properties. Magnetic elements are traditionally used for several applications; nevertheless, they exhibit some limitations such as a low hardness, which must be overcome using elements including B. The hardness of some elements, such as Fe, has been considerably increased by treatment with B
[6]. Recent studies on B transition metal compounds have revealed improved mechanical properties for these compounds
[28]. Due to the presence of unpaired d or f orbitals, magnetic properties such as high coercivity, high magnetization saturation, etc., can be greatly induced in magnetic boron compounds
[29]. A superior magnetism is observed in metallic elements when they are incorporated with borides
[30].
Metallic boride compounds including FeB, NiB, and Co
3B are intermediates between soft and hard magnets. Although they are magnetically hard, they possess low moments; hence, they are considered semi-hard compounds with immense application possibilities in various fields, for example, data storage and biomedicine
[31]. However, very few studies have reported on the development of these compounds as compared to those for other classes of compounds.
2.5. Nonmetallic Non-Oxide System
Nonmetallic non-oxides, such as nitrides, are attracting interest for the induction of magnetization using various elements, similar to the cases of oxides. The magnetism in these compounds is caused by significant spin-exchange interactions in the ionic states. Nevertheless, it is highly useful to stabilize the hole carrier density for doping interactions. Localized acceptors can be utilized to stabilize the quantum confinement effect
[32].
These classes of ferromagnetic compounds are specifically prepared from Group 3 and Group 5 elements, and the thicknesses of GaAs, GaN, InAs, and GaP are inversely proportional to their magnetic saturations; furthermore, these compounds can be fabricated using Group 4 elements such as Ge (for example, Mn
2+-doped Ge). In Group 4 element-based ferromagnetic compounds, ferromagnetism originates from Mn-induced charge carriers, that is, holes in the material matrix. Bound magnetic polarons (BMPs) are formed during exchange interaction between the magnetic dopant and localized holes. These BMPs are composed of magnetic ions and a localized hole, and the interaction of these BMPs in the presence of a high concentration of dopants leads to ferromagnetism
[33]. However, at low temperatures, these BMPs overlap each other and interact with nearby dopants. Eventually, the alignment of these BMPs causes a ferromagnetic transition. Nevertheless, the resulting ferromagnetism is comparatively lesser than those of similar systems. Comparative studies of the saturation magnetizations, observations, and origins of ferromagnetism of different ferromagnetic materials are presented in
Table 1.
Table 1. Comparative studies of the saturation magnetizations, observations, and origins of ferromagnetism of different ferromagnetic materials.
Materials |
Saturation
Magnetization
(emu/g) |
Observation |
Origin of Ferromagnetism |
References |
Traditional materials |
Fe |
217.9 |
Field-induced change in the magnetic domain |
Interactions between electrons in the outermost d orbitals |
[34] |
Co |
162.7 |
Field-induced change in the magnetic domain |
Interactions between electrons in the outermost d orbitals |
[34] |
Ni |
57.5 |
Field-induced change in the magnetic domain |
Interactions between electrons in the outermost d orbitals |
[34] |
Magnetite (Fe | 3 | O | 4 | ) |
90.92 |
Less strongly magnetized than the parent materials |
Magnetic domains of parent materials |
[34][35] |
Maghemite (Fe | 2 | O | 3 | ) |
84–88 |
Less strongly magnetized than the parent materials |
- |
[34] |
CoFe | 2 | O | 4 |
~75 |
Although the parent materials are ferromagnetic, it shows less ferromagnetism |
Magnetism of parent materials |
[34] |
Hexaborides of alkaline-earth metals |
CaB | 6 | films |
Thickness: 0.5 µm (~4.63)
Thickness: 1.6 µm (~0.46)
Thickness: 2.3 µm (~0.102) |
Saturation magnetization is inversely proportional to thickness |
Defects induced by grains boundaries and lattice distortion |
[36] |
CaB | 6 | crystals |
~0.0489 |
Samples demonstrated ferromagnetism |
Surface contamination |
[37] |
BaB | 6 | thin films |
~2.454
at 450–550 | °C |
No variation due to thickness |
Surface contamination |
[9] |
SrB | 6 |
0.06 μ | B | per unit cell |
Temperature affected the magnetic properties |
Defects of surface layers |
[38] |
Nonmagnetic oxides |
HfO | 2 | films |
~13.223 |
Annealing and vacuuming influenced ferromagnetism |
Porous structure of the film
O vacancies |
[39] |
ZnO thin films |
annealed at 150 | °C: |
0.08
annealed at 600 | °C: |
0.42
(at 300 K) |
Thermal annealing under an Ar flow caused a defect |
Single occupied O vacancies |
[40] |
ZnO nanowires |
0.41
at 300 K |
Structural elongation was determined by an applied parallel magnetic field |
2p orbitals of O;
when Zn affects the local spin moment of the O orbital |
[30] |
ZnO films doped with K |
0%K-doped ZnO films: 0.79
4%K-doped ZnO films:
1.09
6%K-doped ZnO films:
1.3
8%K-doped ZnO films:
1.91
11%K-doped ZnO films:
0.63
(at | T | = 300 K) |
With an increase in the K concentration, the saturation magnetization initially increased and then decreased |
Holes
and ZnK defect |
[41] |
ZnO nanoparticles (NPs) |
Raw NPs: Diamagnetic
50 h-milled NPs:
0.031
100 h-milled NPs:
0.047
200 h-milled NPs:
0.086
(at | T | = 300 K) |
Mechanical milling of diamagnetic ZnO powders induced defects.
With an increase in the defect concentration, ferromagnetism increased |
Intrinsic defects related to O and Zn vacancies |
[42] |
|
500 °C | -sintered: 0.0183
| 850 °C | -sintered: 0.0190
| 1300 °C | -sintered: 0.00188
(at | T | = 300 K) |
With an increase in temperature, the saturation magnetization initially increased and then again decreased |
-Interstitial (Zn/O) ion defects
in the samples |
[43] |
ZnO single crystals |
0.63 × 10 | −4 |
(untreated sample)
0.16 × 10 | −3 |
(treated sample)
( | T | = 300 K) |
With an increase in the purity of the sample, the saturation magnetization increased |
O vacancies generated by thermal annealing under an Ar flow |
[30] |
TiO | 2 | films on Si substrates |
PO | 2 | = 50 mTorr: Diamagnetic
PO | 2 | = 0.2 mTorr: Very weakly Diamagnetic +
FM
(~0.005
PO | 2 | = 0.02 mTorr:
~0.075
(At | T | = 25 | °C | ) |
The magnetic moment of the system was inversely proportional to the concentration of O vacancies |
O vacancies |
[44] |
TiO | 2 | films |
Anatase film: ~0.52
Rutile film:
~1.42 |
Using vacuum, O vacancies can be filled |
- Rutile films demonstrated ferromagnetism owing to O vacancies |
[45] |
Anatase TiO | 2 | (12 h H | 2 | -annealed to 873 K) |
0.066 |
Hydrogenation generated local 3d moments |
Complexes of Ti | 3+ | and O defects
Hybridization of O vacancies with Ti 3d–O 2p orbitals |
[46] |
Transition metal ion (TM = Cr, Mn, Fe, Co, Ni, Cu)-doped rutile TiO | 2 | single crystals |
Undoped TiO | 2 | : 0.00016
Cr-doped TiO | 2 | : 0.00036
Mn-doped TiO | 2 | :
0.00055
Fe-doped TiO | 2 | :
0.00136
Co-doped TiO | 2 | :
0.00021
Ni-doped TiO | 2 | :
0.00086
Cu-doped TiO | 2 | :
0.00015 |
Results suggest a close superposition of paramagnetic and ferromagnetic behaviors |
Separation of the metallic phases of Ni, Co, and Fe
Unpaired d electrons of transition metal ions |
[47] |
CeO | 2−x | films |
When x = 0.03:
~1.34
When
x = 0.1:
~1.02
( | T | = 300 K) |
Both Ce | 3+ | and Ce | 4+ | are present |
O and Ce vacancies |
[48] |
MgO films |
Untreated sample: ~0.751
Annealed sample: ~0.329 |
Reduction in the concentration of Mg vacancies is proportional to the reduction of Mg after annealing |
Mg cation vacancies |
[49] |
ZrO | 2 | with Fe |
205.56 |
Analysis helped to improve the magnetic characteristics of this system |
Induced defects and stress |
[50] |
High-purity SnO | 2 | powders |
0 h-milled:
0.0006
4 h-milled:
0.0019
12 h-milled:
0.0055
20 h-milled:
0.0105 |
Temperature increases inversely with saturation magnetization |
Singly charged O vacancies
High defect density
- |
[51] |
SnO | 2 | NPs |
Powder in raw form: 0.019
Powder annealed at
773 K:
0.015
Powder annealed at
973 K:
0.012
Powder annealed at
1173 K:
0.010
Powder annealed at 1373 K:
0.006
Powder annealed at
1573 K:
0.001
( | T | = 300 K) |
The saturation magnetizations of NPs reduced when the NPs were annealed at temperatures higher than 500 °C |
O vacancies ( | T | = 5 K) |
[52] |
Carbon Nanostructures |
Highly oriented graphite samples |
Kish graphite:
0.6 × 10 | −3 | ± 0.2 × 10 | −3 |
at | T | = 300 K |
Different possibilities for the ferromagnetic-like behaviors in the samples |
Magnetic impurities
Topological defects
Itinerant ferromagnetism |
[53] |
C | 60 |
0.045
| (T-A=πr2527 °C) A=πr2 |
Upon applying a pressure of 9 GPa at 800 K, the ferromagnetic behavior significantly decreased |
C radical formation |
[54] |
Graphene |
Annealing at | T | = 300 | °C |
At 300 K: 0.004
At 2 K: 0.25
Annealing at | T | =
−500 | °C |
At 300 K: 0.020
At 2 K: 0.90 |
Graphene prepared at 1073 K
did not clearly exhibit ferromagnetism |
Defects induced by annealing |
[27] |
Graphene nanoribbons |
1.1 |
Optimization of density twist and turn edge defects |
Defect density |
[55] |
Implantation of ions on pyrolytic graphite—12C |
14.4 |
Implantation steps are directly proportional to the vacancy density |
Vacancy density |
[56] |
C Nanotubes |
0.5227 |
N | 2 | plasma treatment |
Amine- and N pyridine-based bonding configuration |
[57] |
Magnetic Borides |
Ni | 2 | B with O |
29 |
Treatment of Ni with boride prevented the oxidation of Ni |
Intrinsic defects
- |
[58] |
CoB |
75–135 |
Change in magnetic properties with an increase in crystallization |
Intrinsic defects |
[59] |