Residential and commercial buildings are responsible for over 30% of global final energy consumption and accounts for ~40% of annual direct and indirect greenhouse gas emissions. Energy efficient and sustainable technologies are necessary to not only lower the energy footprint but also lower the environmental burden. Many proven and emerging technologies are being pursued to meet the ever-increasing energy demand. Catalytic science has a significant new role to play in helping address sustainable energy challenges, particularly in buildings, compared to transportation and industrial sectors. Thermally driven heat pumps, dehumidification, cogeneration, thermal energy storage, carbon capture and utilization, emissions suppression, waste-to-energy conversion, and corrosion prevention technologies can tap into the advantages of catalytic science in realizing the full potential of such approaches, quickly, efficiently, and reliably. Catalysts can help increase energy conversion efficiency in building related technologies but must utilize low cost, easily available and easy-to-manufacture materials for large scale deployment. This entry presents a comprehensive overview of the impact of each building technology area on energy demand and environmental burden, state-of-the-art of catalytic solutions, research, and development opportunities for catalysis in building technologies, while identifying requirements, opportunities, and challenges.
1. Introduction
Energy plays a vital role in modern society; however, it is also responsible for greenhouse gas emissions. Global energy consumption is on the rise driven by economic and population growth. As shown in Figure 1, although renewables are expected to become the primary resource, fossil fuels continue to increase, given the global demand increase [1].
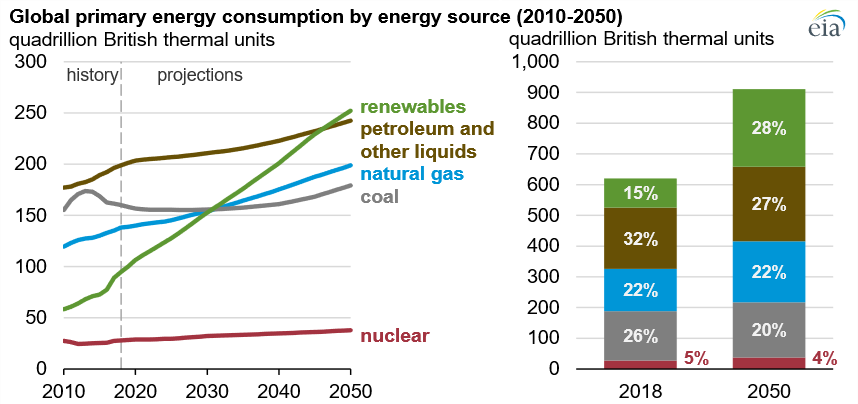
Figure 1.
Global primary energy consumption by energy source, quadrillion Btu
.
By year 2050, energy information administration (EIA) projects a 50% increase in global energy demand driven by a 65% increase in building energy consumption, a 79% increase in electrical power generation, and a 40% increase in natural gas consumption
[2]. A continuous upsurge in energy demand due to increased affordability, economic growth, and new energy consumers poses a significant challenge to the energy infrastructure and the environment. Three major energy-consuming sectors in any economy are buildings, transportation, and industry. Amongst these energy consumers, residential and commercial buildings account for more than 30% of primary energy consumption. Combined direct and indirect carbon footprint of the buildings sector approached almost 40% of total carbon dioxide emissions
[3]. According to energy information administration statistics
[4], residential and commercial buildings in the USA consumed 41 exajoules (1.3 terawatt-year) of energy in the year 2019, accounting for almost 39% of total primary energy. As shown in
Figure 2, the electricity supply to the end consumer in the USA comes at a premium value since ~65% of the primary energy is lost in the process of production and distribution.
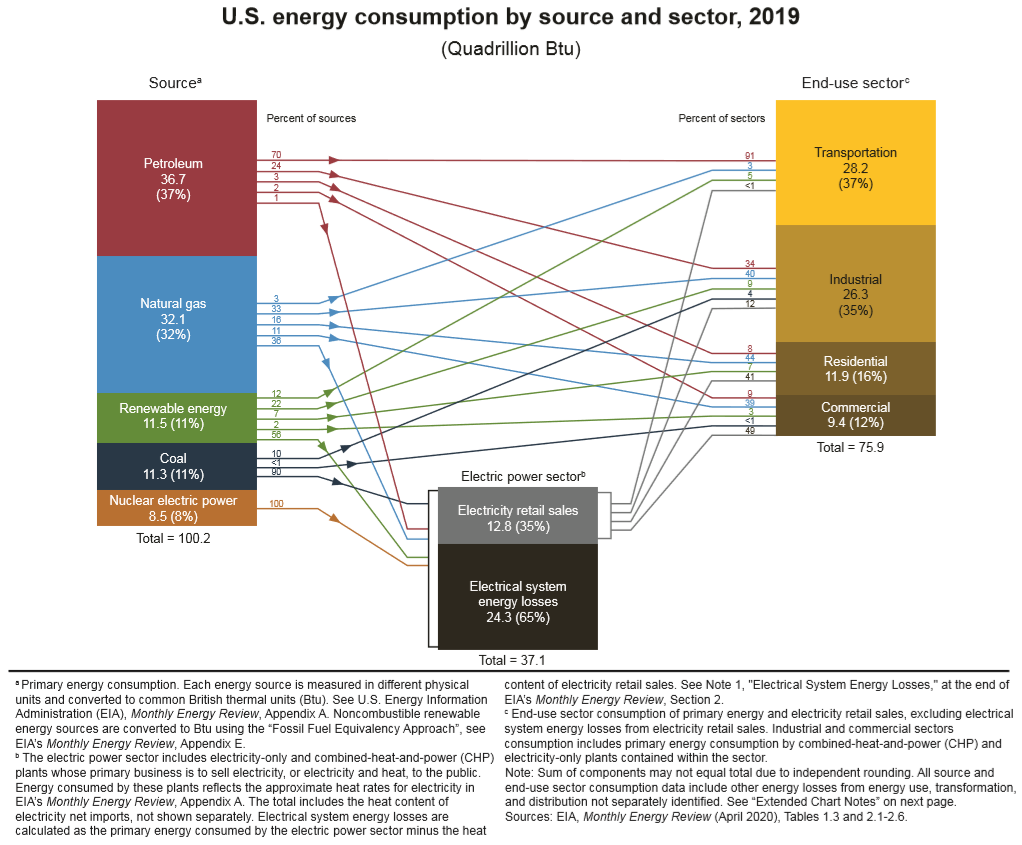
Figure 2. U.S. Energy consumption by source and sector
[4].
a Primary energy consumption. Each energy source is measured in different physical units and converted to common British thermal units (Btu).
b The electric power sector includes electricity-only and combined-heat-and-power plants.
c End-use sector consumption of primary energy and electricity retail sales, excluding electrical system energy losses from electricity retail sales.
Given this high premium, it is essential that the electric energy supplied to buildings is utilized to its full potential at the highest possible conversion and utilization efficiency. From an energy efficiency perspective, heating and cooling equipment in a residential or commercial building consumes up to 50% of the total energy supply
[5][6] in the USA. Heating systems, for instance, utilize ~60% fossil fuels as the primary energy resource
[7] in the USA, as shown in
Figure 3. As a result, building energy usage accounts for ~35% of total annual carbon dioxide emissions in the USA
[8], as shown in
Figure 4.
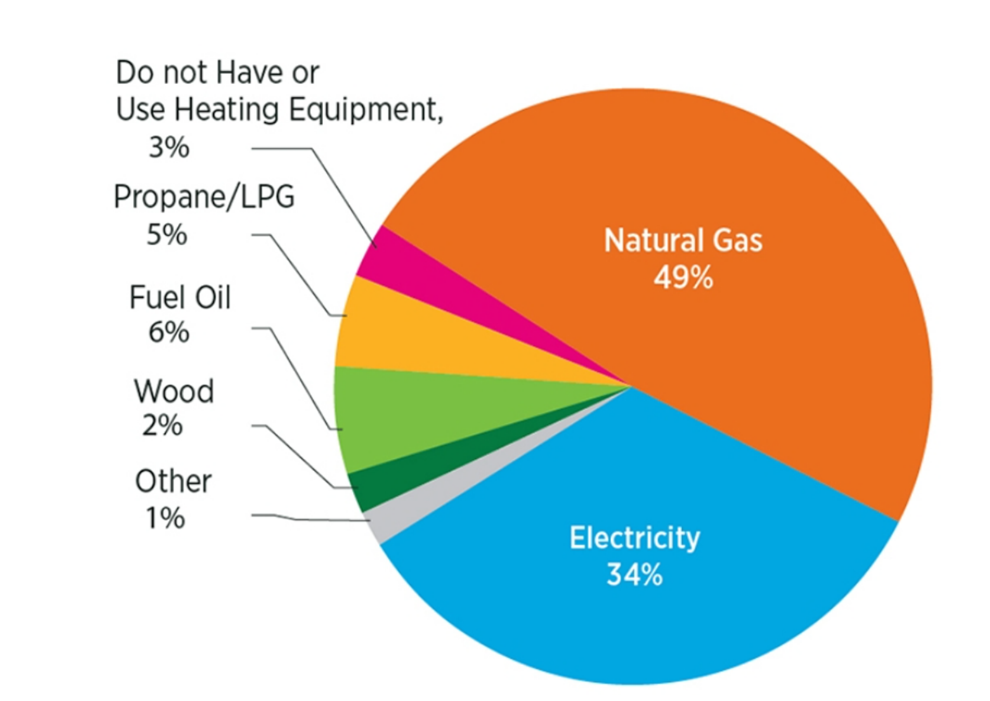
Figure 3.
U.S. household heating systems’ energy source
[7]
.
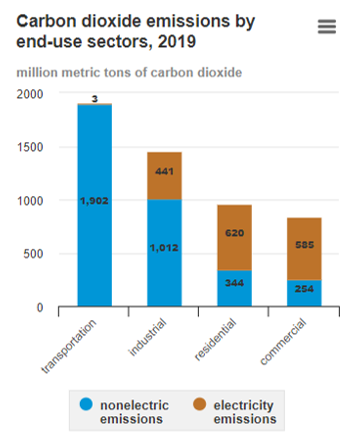
Figure 4.
U.S. carbon dioxide emissions from energy consumption by sector
.
Given these energy and environmental impacts and projected statistics, technology improvements to address high energy demand, carbon footprint, and emissions associated with building energy consumption is of enormous value for a sustainable energy future. Additionally, buildings offer great potential to improve quality of life, health, and workforce productivity (defined as an assessment of the efficacy of worker(s)). For instance, a recent study by national institute of health
[9] focusing on the relationship between carbon dioxide, volatile organic compounds (VOC) concentrations, and associated the impact of air quality on cognitive function scores in office environments. The authors looked at nine different cognitive function domains as a function of carbon dioxide and VOC concentrations and found that clean and green buildings improve the scores by 50% to 200% depending on the activity domain.
Similarly, indoor air quality improvements are highly essential for the improved health of occupants in buildings. Various studies have established the significance of pollutant concentrations in homes and their impact on health and productivity of residents
[10]. A review of interactions between energy performance of the buildings, outdoor air pollution and the indoor air quality was recently reported
[11][12]. These studies investigated the impact of building energy efficiency improvements on pollutant concentrations and their health risk.
Increase in energy demand coupled with intermittent renewable energy resources in the grid infrastructure also emphasizes the importance of energy management and flexibility. For instance, efficient use of energy resources focusing on grid resiliency and environmental security supported by cogeneration and trigeneration technologies is a highly impactful approach. Numerous studies have already established the significance of such systems
[13][14]. These behind-the-meter assets have a significant potential in enabling sustainable energy technologies and can be deployed across the grid. Considering these factors, many opportunities exist in improving the health, productivity, energy efficiency, carbon footprint, and energy management for a sustainable future. Building energy resources such as heating, cooling, dehumidification, and thermal storage equipment, along with carbon capture/conversion, cogeneration and biomass conversion technologies play a vital role in realizing energy sustainability, occupant health and productivity, and environmental responsibility. In addition, energy efficiency, affordability, and retrofittability are some of the key attributes necessary for successful transition towards new technologies.
All these technologies utilize a vast array of chemical transformations, including (i) oxidation—for instance, pollutants, fuel conversion to syngas, and thermal storage, (ii) reduction—carbon dioxide conversion, (iii) hydration—thermal storage, (iv) dehydration—dehumidification, (v) absorption/chemisorption—heating and cooling, carbon capture, and (vi) hydrolysis and methanogenesis—biomass conversion to biogas. Given these chemical reactions and their potential for enabling sustainable societies, catalysis has a new significant role to play in buildings. In this context, the objective of this entry is to provide a comprehensive overview of the state of the art of catalysts in building applications, their impact, and future research and development opportunities. The next section introduces individual technology areas followed by a discussion on future directions for catalysts in enabling clean and sustainable energy in buildings.