African swine fever virus (ASFV) is the causative agent of the epidemic of African swine fever (ASF), with virulent strains having a mortality rate of up to 100% and presenting devastating impacts on animal farming. ASFV is the only known arbovirus in the Nucleoplasmic Large DNA Virus (NCLDV) family. It has an icosahedral structure and envelope with a diameter of 200nm. The length of the genome varies between 170 and 190 kbp, encoding 151 to 167 open reading frames (ORF), which are closely spaced along two strands of viral DNA and separated by short intergenic regions.
1. Introduction
In 1921, A
frican swine fever virus (ASFV
) was first reported in Kenya, which caused about an 100% mortality in infected domestic pigs
[1]. Subsequently, ASFV spread to most African countries south of the Sahara
[2]. In 1957 and 1960, the African swine fever epidemic broke out in Portugal which was the first time to demonstrate that ASFV spread across continents and spread to other European countries, the Caribbean, and Brazil
[3]. Except in Sardinia, the genotype I ASFV was eradicated in the 1990’s
[4]. However, the genotype II ASFV was introduced to Georgia in the Caucasus region and started a new round of transmission in 2007
[5]. Afterwards, it spread to the Russian Federation, Ukraine and Belarus, and in 2014 it spread to Eastern European countries
[4][5][4,5]. In 2018, the epidemic spread to Belgium, Hungary, Czech Republic, Romania, Bulgaria, Slovakia, and Serbia, as well as China and other parts of Asia (Mongolia, Korea, Vietnam, Laos, Cambodia, Myanmar, the Philippines, Hong Kong, and Indonesia)
[3]. ASF outbreaks are still trending regionally, with new regional reports of ASF outbreaks. In September 2019, Timor-Leste reported the first outbreak of African swine fever in Oceania, followed by Papua New Guinea (March 2020)
[6][7][6,7]. In July 2021, ASF reappeared in the Americas, first in the Dominican Republic and later in Haiti
[8][9][8,9]. In January 2022, ASF genotype II was notified in mainland Italy
[9]. In January 2022, two new countries also reported their first cases of the disease: North Macedonia in Europe and Thailand in Asia
[9]. According to OIE, from January 2020 to January 2022, ASF outbreaks were reported in 35 countries or regions around the world, including 4767 cases (1043334 animals lost) in domestic pigs and 18,262 cases (29970 animals lost) in wild boars
[9]. It is worth noting that wild boar cases in Europe accounted for the vast majority (83.3%, 16743/20107), while wild boar cases in Asia accounted for a lower proportion of 59.4% (1519/2559)
[9]. The wild boar reservoir of ASFV will present challenges to the eradication of ASF by vaccination programs.
In August 2018, the first ASF case in China was identified in Shenyang, Liaoning Province
[10]. Since most pig farms in China are small-scale pig farms with very low biosecurity levels, the rapid spread of ASFV across the country has led to a serious reduction in the number of live pigs and caused heavy losses to the pig industry
[11]. The decline in the number of live pigs has led to a rapid rise in the price of pork in a short period of time, affecting the normal life of residents in China. After the outbreak of ASF, with the joint efforts of Chinese government leaders and industry practitioners, various pig farms tried to resume production. By improving the level of biosecurity, including optimizing the location of pig farms, strict disinfection measures, and strengthening ASFV testing on pig farms, ASFV-positive pigs were culled at designated locations, and the source of the infection was eliminated. Most pig farms finally successfully resumed breeding
[12]. However, in order to improve the level of biosecurity, the measures taken by pig farms have objectively increased the cost of breeding. Therefore, there is an urgent need for vaccines for the effective control and eradication of ASF.
Hemadsorption (HAD) refers to the ability of ASFV-infected macrophages to adsorb erythrocytes, and non-hemadsorption (non-HAD) refers to the inability of ASFV-infected macrophages to adsorb erythrocytes. ASFV has spread for more than three years in China, and its epidemic trend has become more complicated with the emergence of non-hemadsorbing (non-HAD) naturally attenuated strains of ASFV
[13][14][15][13,14,15]. The initial clinical symptoms of domestic pigs infected with low-viral strains are not obvious, which is difficult to diagnose
[13][14][13,14]. Comparative analysis showed that the genome of the low-virulence strains had mutations, deletions, insertions or substitutions of short fragments, including the deletion or mutation of the CD2v (EP402R) gene, which is related to the HAD phenotype
[16]. In addition, genotype I strains have also been reported in China (
Figure 1)
[14]. The potential for endemic ASF outbreaks is increasing due to the widespread distribution of wild boars in China. Researchers are actively conducting ASF vaccine-related research, including the evaluation of live attenuated vaccines (LAVs) vaccines by knocking out virulence genes, and the screening of antigens with protective effects to develop subunit vaccines, vector vaccines, etc.
[17][18][19][20][21][22][23][24][25][26][27][28][29][30][17,18,19,20,21,22,23,24,25,26,27,28,29,30]. ASFV has evolved the ability to manipulate host immune responses by encoding many immune escape genes, including regulation of IFN expression, inhibition of autophagy, and apoptosis. In addition, ASFV affects the antigen presentation and activation of lymphocytes by invading monocytes-macrophages and DCs, thereby affecting the immune system of the entire body
[31].
Figure 1. A phylogenetic tree was constructed based on the whole genome sequences of 74 strains in the GenBank database, after which datasets for the sequences were aligned using MAFFT (version 7.149) program
[32]. Maximum likelihood (ML) phylogenies for the codon alignment of the genome sequences were estimated using the GTRGAMMA nucleotide substitution model in the IQ-TREE 1.68 software
[33]. Node support was determined by nonparametric bootstrapping with 1000 replicates, and the phylogenetic tree was visualized in the Figtree (version 1.4.3) program (
http://tree.bio.ed.ac.uk/software/Figtree/) (accessed on 18 January 2022). Types written in red indicate Chinese isolates.
2. The Major Defense Mechanisms and African Swine Fever Virus (ASFV) FV Interactions
2.1. Physical Barriers
The skin barrier is the most effective physical defense of the host to block entry of pathogens. The physical barriers of the body’s defenses include intact skin, surfaces of the respiratory and gastrointestinal tracts and include processes of self-cleaning (coughing, sneezing and mucus flow in the respiratory tract, vomiting, diarrhea, and urination of the urinary system, etc.), and normal flora on the skin surface and in the gut. The transmission of ASFV among pigs is mainly direct contact transmission, including transmission through mouth-nose contact and short-distance aerosol transmission
[34]. In addition, the presence of soft tick bites in pig farms can also cause the transmission of ASFV
[35]. Therefore, it is necessary to improve animal welfare in the farm, and increase the resistance of animals by reducing the breeding density. Pig farms should strengthen environmental sanitation and disinfection to block the spread of the virus. On the contrary, for infected animals, the self-cleaning of the body is also conducive to virus spread. It is necessary to regularly collect and detect the presence of ASFV in pig herds, and to ensure the removal and safe destruction of positive pig herds to eliminate the source of infection
[12].
2.2. Innate Immunity
Sentinel cells, such as macrophages, dendritic cells, and mast cells, elicit inflammatory responses by recognizing pathogen-associated molecular patterns (PAMPs) of microorganisms through pattern recognition receptors. The inflammatory response involves the activation and directed migration of various cells from the blood to the site of invasion, especially neutrophils and macrophages. The pattern recognition receptors that recognize ASFV mainly include TLR3 that recognizes viral dsRNA and cGAS-STING that recognizes viral DNA in the cytoplasm
[36][37][38][36,37,38]. When TLR3 binds to dsRNA, it transmits signals to cells, resulting in increased expression of nuclear factor kappa-B (NF-κB), which in turn activates IL-1, IL-6 and TNF-α and other cytokine expression
[36]. The cGAS-STING system recognizes virus DNA, and the virus-infected (mainly plasmacytoid dendritic cells) cells produce IFN-I
[38]. IFN-I acts on virus-infected cells to inhibit virus growth.
ASFV has also acquired many genes in evolution that can regulate the inflammatory response in the early stage of the body. ASFV-encoded genes also primarily inhibit the actions of TLR3 and the cGAS-STING system (
Figure 2). Studies have shown that the I329L and A276R proteins encoded by ASFV have immunosuppressive effects
[39][40][39,40]. I329L (the homologous protein of TLR3) interacts with the adaptor protein TRIF to inhibit the activation of NF-κB and IRF3, while A276R can also inhibit the recognition of TLR3
[37][39][37,39]. In addition, another study showed that TLR expression was decreased after ASFV infection of macrophages
[40].
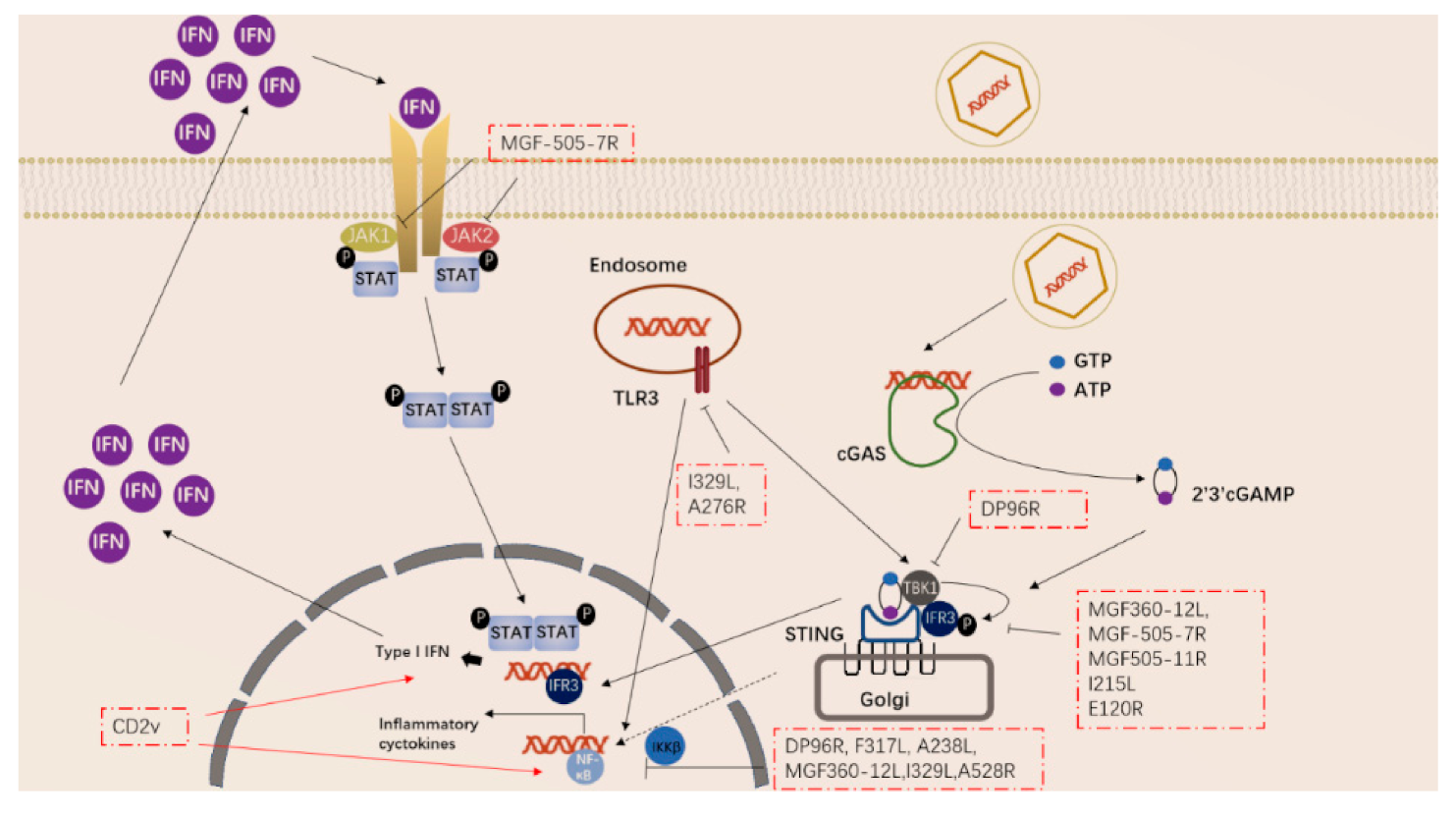
Figure 2. ASFV genes regulate innate immune signaling pathway.
ASFV encodes multiple genes involved in suppressing the cGAS-STING signaling pathway. In 2013, Correia et al. reported that A528R (MGF530) could inhibit the expression of IFN-β by targeting IRF3 and NF-κB or through the JAK-STAT pathway
[39][41][39,41]. In 2018, Wang et al. found that DP96R targets TBK1 and IκB kinase beta (IKKβ) to negatively regulate IFN-I expression and induction of NF-κB signaling
[42]. In 2020, Zhuo et al. demonstrated that MGF360-12L can block the interaction between p65 and KPNA2, KPNA3, and KPNA4, and can interfere with the nuclear translocation of NF-κB
[43]. Five immunosuppressive genes were reported in 2021, including E120R, F317L, MGF505-7R, MGF505-11R, and I215L
[44][45][46][47][48][49][44,45,46,47,48,49]. E120R can interact and block the activation of IRF3, thereby inhibiting the expression of IFN-β
[44]. F317L inhibits IKKβ phosphorylation, thereby reducing the phosphorylation and ubiquitination of IkBα, thereby inhibiting the activation of the NF-κB pathway
[45]. Li et al. reported that MGF-505-7R promoted the expression of autophagy-related protein ULK1 to degrade STING, and inhibited IFN-γ-mediated JAK1 and JAK2-mediated signaling pathways
[46][48][46,48]. Similarly, Yang et al. reported that MGF505-11R interacts with STING, degrades STING expression through lysosome, ubiquitin-proteasome and autophagy pathways, and can inhibit the phosphorylation of TBK1 and IRF3 stimulated by cGAS/STING overexpression
[47]. pI215L recruits RNF138 to inhibit K63-related TBK1 ubiquitination, which has an inhibitory effect on IFN-I production
[49]. It is worth noting that CD2V can activate NF-κB and induce activation of IFN signaling pathways in porcine lymphocytes/macrophages
[50]. These immunosuppressive genes are potential target genes for the preparation of live attenuated vaccines.
3. ASF Vaccines
3.1. Live Attenuated Vaccines (LAVs)
LAVs can generally be divided into natural attenuated strains and artificial gene deletion strains, and the latter is made by deleting certain virulence genes. Compared with other types of ASF vaccines, LAVs can provide complete homologous and partial heterologous protection
[51][79]. The most promising LAVs vaccine candidates are summarized in
Table 1. Chen et al. reported that a seven-gene deletion LAV had a good protective effect
[17]. In addition, Zhang et al. reported that deleting L7L-L11L attenuates ASFV. In vaccination experiments, these attenuated strains conferred 100% protection against homologous challenge
[18]. LAVs have experienced a trend from multiple gene deletions to single gene deletions. Protection induced by immunization with LAVs correlates with the level of replication of the LAVs in addition to the number of immunogenic genes expressed. If the replication of LAVs is severely attenuated, then the LAVs will not be immunogenic. LAVs with single gene deletions express more genes and may cause better protection. ASFV-G-∆I177L could achieve 100% protection through oral and injection routes, and field trials have been completed in Vietnam
[19][20][21][19,20,21]. Similarly, ASFV-G-ΔA137R and SY18ΔI226R were proven to confer 100% protection
[22][23][22,23].
Table 1.
Summary of the most promising LAV candidates.
Genes |
Strains |
Genotype |
Minimal Protective Dose |
Route |
Challenge |
Gene Function |
References |
I177L |
Georgia2007/1 |
II |
10 | 2 | HAD | 50 |
IM |
Georgia2007/1 |
unknown |
[19] |
10 | 6 | HAD | 50 |
ON |
Georgia2007/1 |
[20] |
10 | 2 | HAD | 50 |
IM |
TTKN/ASFV/DN/2019 |
[21] |
A137R |
Georgia2007/1 |
II |
10 | 2 | HAD | 50 |
IM |
Georgia2007/1 |
unknown |
[23] |
I226R I226R |
SY18 |
II |
10 | 4 | HAD | 50 |
IM |
SY18 |
unknown |
[22] |
L7L-L11L |
SY18 |
II |
10 | 3 | HAD | 50 |
IM |
SY18 |
unknown |
[18] |
MGF505/360(6) | 1 | and EP402R |
HLJ/18 |
II |
10 | 3 | HAD | 50 |
IM |
HLJ/18 |
hemadsorbing and inhibition of type I interferon responses |
[17] |
10 | 5 | HAD | 50 |
ON |
EP402R |
Ba71V |
I |
10 | 4 | HAD | 50 |
IM |
Ba71V |
hemadsorbing |
[52] | [86] |
E75 |
Georgia2007/1 |
Historically, attenuated strains were once used in Spain and Portugal, but widely caused chronic ASF infections in vaccinated pigs. Therefore, adequate experiments should be conducted to verify the effectiveness and safety of LAVs before extensive promotion, for example, future ASF vaccines should be distinguish between infected animals and vaccinated animals (DIVA)
[53][80]. Ramirez-Medina et al. constructed a deletion strain of E184L to distinguish between infected animals and vaccinated animals (DIVA), but the deletion strain cannot provide complete protection
[53][80]. LAVs are still far from commercialization due to the safety issue. LAVs may have the risk of virulence reversion during large-scale vaccination. Therefore, adequate experiments should be conducted to verify the effectiveness and safety of LAVs before extensive promotion.
In addition, the lack of cell lines for large-scale LAVs production is another difficulty. ASFV subculture can adapt to cell lines such as 293 and Vero, but the virulence and antigenicity of the adapted strains are weakened, and adapted strains cannot provide effective protection after immunizing pigs
[54][55][81,82]. The adapted cells cultured in LAVs are primary cells, mainly including porcine alveolar macrophages (PAM) and porcine bone marrow cells (PBMS). Primary cells are not sufficient for large-scale production of LAVs. Borca et al. reported that ASFV-G-ΔI177L/ΔLVR could replicate efficiently in stable porcine cell lines with potent protection
[56][83]. In addition, Takenouchi et al. reported a novel immortalized porcine macrophage cell line, IPKM, which was generated by transduction of primary PKM with lentiviral vectors encoding SV40LT and pTERT
[57][84]. However, it is necessary to carefully test it before using IPKM as a production cell line for a live-attenuated vaccine strain.
Finally, the cross-protective ability of LAVs needs to be further evaluated. ASFV has mutations, duplications, and loss of certain sequences in the genomes of different genotypes or even different strains of the same genotype, which may lead to changes in virulence
[58][85].
3.2. Subunit Vaccines
Subunit vaccines are generally in the laboratory research stage, and there is still a huge gap between practical applications. It has been confirmed that some ASFV antigens have a certain protective effect
[24][26][24,26]. Antibodies against p72 and p54 can block the adsorption of ASFV, and antibodies against p30 can block the internalization of the virus
[59][87]. The key to subunit vaccines is to screen for those antigens or those epitopes of antigens that have definite protective effects
[60][88]. In the presence of non-neutralizing antibodies, the virus-antibody complex formed is infectious. The virus bound by the non-neutralizing antibody is phagocytosed by macrophages, and the virus can still grow in the macrophage at this time, and the virus bound by the non-neutralizing antibody accelerates the replication of the virus
[61][62][89,90].
Previous studies have noted a critical role for CD8
+ T cells in protection, as demonstrated by using the virulent Portuguese ASFV isolate OUR/T88/1
[63][91]. DNA vaccines as well as vector vaccines have been attempted as alternative ASF vaccine platforms. In theory, DNA vaccines as well as vectors have better immunogenicity because the antigen can be expressed intracellularly and via MHC I, which is important for CD8
+ T cell activation. Neither DNA vaccine, vector vaccine, nor prime-boost immunization strategies can provide complete protection
[64][65][66][67][56,92,93,94]. Luckily, Goatley et al. cloned B602L, p72, p30, p54, E199L, EP153R, F317L, and MGF505-5R into adenovirus vectors for primary immunization, and into MVA vectors for booster immunization, then found the protective function up to 100% in pigs
[30]. However, low levels of infectious virus remained in the immunized pigs until the end of the trial
[30]. Future experiments should determine whether pigs can clear the infection and whether these vaccinated animals shed infectious virus
[30].
Similar to screening for antigens for neutralizing antibodies, screening is also required to identify antigenic epitopes capable of inducing ASFV-specific T cells. Bosch-Camós used in silico prediction of ASFV protein CD8
+ T cell epitopes and predicted a 19-mer peptide from MGF100-1L
[68][76]. Netherton et al. predicted peptides corresponding to 133 proteins encoded by OUR T88/3, and screened 18 viral proteins that could be recognized by lymphocytes of ASF-immunized pigs
[69][78]. The specific antigenic epitopes are determined by software analysis and verification in combination with relevant experiments, which guides the selection of antigens in the development of ASF vaccines.