1. Introduction
Huntington disease (HD) is an autosomal, dominant, lethal neurodegenerative disorder affecting between 0.42 and 17.2 per 100,000 individuals around the world [1,2,3] HD results in a wide range of symptoms, including involuntary movements, clumsiness, lack of concentration, memory lapses, mood swings, and depression. Although brain pathology is considered a hallmark of HD, new studies suggest that peripheral tissue pathology is an important factor in disease manifestation and progression. In particular, HD mouse models have recently been shown to display skeletal muscle malfunction and HD-related cardiomyopathy [4,5,6].
Primary symptoms generally occur in adults (typical age range: 40–45 years) and gradually progress, leading to the deterioration of patients’ health, and finally death, after 10–20 years. Although several clinical trials are ongoing, there is no cure for HD at present. HD is caused by a mutation in the HTT gene, which encodes for the huntingtin (Htt) protein. The HTT gene contains a repeat of 6–35 CAG triplets in exon 1, which is translated into a polyglutamine (polyQ) stretch in the Htt N-terminal region. The HD patients’ gene contains ≥36 CAG triplets, encoding for a mutated Htt (mHtt) with an expanded polyQ region [7]. mHtt is highly susceptible to aggregation with other mHtt molecules or different proteins, leading to the formation of clusters, fibrils and inclusions, some of which comprise 100,000s of mHtt molecules and are large enough to be visualized by light microscopy [8,9].
CAG stretches that are longer than 60 repeats in the Htt gene are associated with Juvenile-onset Huntington Disease (JoHD). This is a rare HD variant that accounts for about 4–10% of all cases, typically defined based on the appearance of symptoms at age 20 years or younger [10]. JoHD patients experience different motor and non-motor symptoms at disease onset and throughout the disease course, with a faster disease progression rate and reduced life span with respect to adult HD patients [11].
Since both soluble and aggregated mHtt are well known to induce ER (Endoplasmic Reticulum) stress, leading to neuronal injury and apoptosis [12], both the inhibition of mHtt aggregate formation and the acceleration of mHtt degradation could be exploited for HD symptom delay, or even treatment [13]. mHtt can be degraded by two main pathways: the ubiquitin–proteasome system (UPS) and the autophagy–lysosomal pathway. In this paper, we focus on the UPS, since it has been shown to play a more important role in removing mHtt than the autophagy–lysosomal pathway [14]. In this framework, it is important to underline that cells contain a variety of molecular chaperones and other proteins, such as heat shock family proteins HSP40, HSP70, HSP90, and HSP105, which are able to identify and combine with misfolded mHtt to inhibit aggregate formation to different degrees, leading to cell survival [15,16,17,18,19].
Here, we describe the mechanism of mHtt ubiquitination, with particular emphasis on the structure–function relationships in E3 ligases involved in the process. Finally, we discuss how knowledge of these relationships can be exploited to develop PROteolysis TArgeting Chimeras (PROTACs), which may be used to develop innovative drugs able to increase mHtt clearance in HD patients.
2. Ubiquitin Proteasome System (UPS)
The UPS comprises ubiquitin (Ub), proteasome, and three classes of enzymes, and plays key roles in various essential biological processes, such as cell cycle progression, signal transduction, maintenance of genome integrity, and tumorigenesis [20].
UPS activity consists of two main processes: (1) covalent attachment of multiple Ub molecules to the target protein; and (2) degradation of the resulting covalent target protein–Ub complex by the 26S proteasome complex (Figure 1.). The first process, in turn, encompasses several steps and requires at least three classes of enzymes: (i) Ub-activating enzymes (E1); (ii) Ub-conjugating enzymes (E2); and (iii) Ub ligases (E3). In the first step, E1 enzymes use ATP to activate the Ub carboxy-terminal region (C-ter). The resulting Ub–AMP drives the formation of a thioester bond between Ub and a cysteine residue of E1. In the second step, Ub is transferred to a cysteine residue of an E2 enzyme. In the third step, the E3 ligase binds both the Ub–E2 adduct and the target protein. In step four, an isopeptide bond between Ub and the protein target is formed (Figure Int1) [21,22]. The process that consists in the linkage of a protein to a single Ub monomer is known as monoubiquitination.
Huntington disease (HD) is an autosomal dominant lethal neurodegenerative disorder affecting between 0.42 to 17.2 per 100,000 individuals around the world[1][2][3]. HD results in a wide range of symptoms, including involuntary movements, clumsiness, lack of concentration, memory lapses, mood swings and depression. Although brain pathology is considered a hallmark of HD, new studies suggest that peripheral tissue pathology is an important factor in disease manifestation and progression. In particular, HD mouse models have been recently shown to display skeletal muscle malfunction and HD-related cardiomyopathy[4] [5] [6].
Primary symptoms generally occur in adults (typical age range: 40-45 years) and gradually progress, leading to deterioration of patients’ health and finally death, after 10-20 years. Although several clinical trials are ongoing, there is no cure for HD at present. HD is caused by a mutation in the HTT gene, which encodes for the huntingtin (Htt) protein. The HTT gene contains a repeat of 6-35 CAG triplets in exon 1, which is translated into a polyglutamine (polyQ) stretch in Htt N-terminal region. The HD patients’ gene contains ≥36 CAG triplets, encoding for a mutated Htt (mHtt) with an expanded polyQ region [7]. mHtt is highly susceptible to aggregation with other mHtt molecules or different proteins, leading to the formation of clusters, fibrils and inclusions, some of which comprise 100,000s of mHtt molecules and are large enough to be visualized by light microscopy [8][9].
CAG stretches longer than 60 repeats in the Htt gene are associated with Juvenile-onset Huntington’s Disease (JoHD). This is a rare HD variant that accounts for about 4–10% of all cases, and is typically defined based on the appearance of symptoms at age 20 years or younger[10]. JoHD patients experience different motor and non-motor symptoms at disease onset and throughout the disease course, with faster disease progression rate and reduced life span with respect to adult HD patients [11].
Since both soluble and aggregated mHtt are well known to induce ER stress, leading to neuronal injury and apoptosis [12], inhibition of mHtt aggregates formation and acceleration of mHtt degradation might be exploited for HD symptoms delay, or even treatment [13]. mHtt can be degraded by two main pathways: the autophagy-lysosomal pathway and the ubiquitin-proteasome system (UPS), which has been shown to play a more important role in removing mHtt than the autophagy-lysosomal pathway [14]. In this framework, it is important to underline that cells contain a variety of molecular chaperones and other proteins, such as heat shock family proteins HSP40, HSP70, HSP90 and HSP105, which are able to identify and combine with misfolded mHtt to inhibit aggregate formation to different degrees, leading to cell survival [15][16] [17][18] [19].
- Ubiquitin Proteasome System (UPS)
The UPS comprises ubiquitin (Ub), proteasome and three classes of enzymes, and plays key roles in various essential biological processes, such as cell cycle progression, signal transduction, maintenance of genome integrity, and tumorigenesis [20].
UPS activity consists in two main processes: 1) Covalent attachment of multiple Ub molecules to the target protein; and 2) Degradation of the resulting covalent target protein-Ub complex by the 26S proteasome complex (Figure 1). The first process, in turn, encompasses several steps and requires at least three classes of enzymes: i) Ub-activating enzymes (E1); ii) Ub-conjugating enzymes (E2); and iii) Ub ligases (E3). In the first step, E1 enzymes, use ATP to activate the Ub carboxy-terminal region (C-ter). The resulting Ub-AMP drives the formation of a thioester bond between Ub and a cysteine residue of E1. In the second step, Ub is transferred to a cysteine residue of an E2 enzyme. In the third step, the E3 ligase binds both the Ub-E2 adduct and the target protein. In step four, an isopeptide bond between Ub and the protein target is formed (Figure 1) [21][22]. The process consisting in the linkage of a protein to a single Ub monomer is known as monoubiquitination.
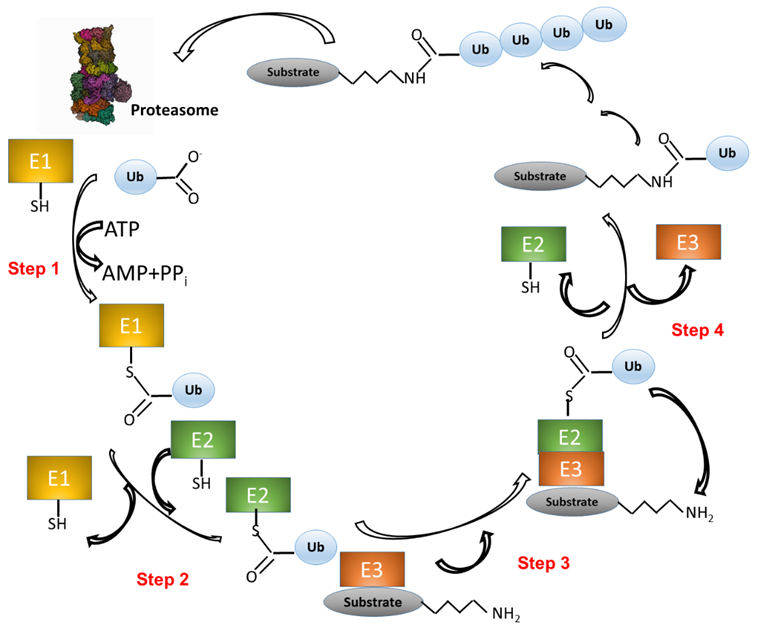
e 1. The ubiquitination pathway. (1) Ubiquitin (Ub) is linked to a cysteine residue of a Ub-activating enzyme (E1) via a reaction that uses the energy derived from the hydrolysis of an ATP molecule. (2) Ub is transferred from E1 to the cysteine of a Ub-conjugating enzyme (E2). (3) A complex is formed between E2–Ub and a Ub ligase (E3), which is able to bind a protein substrate. (4) Ub is transferred from E2 to a lysine residue of the protein substrate. Other Ub molecules can be added to the substrate–Ub complex with the same mechanism (steps 1–4). Finally, the polyubiquitinated substrate is recognized and degraded by the proteasome, whereas Ub is released and ready to start a new cycle.
Once monoubiquitination has occurred, the Ub moiety can be ubiquitinated again at one of the seven lysine sidechains (K6, K11, K27, K29, K33, K48, and K63) or at the free main-chain amine group of the N-terminal methionine residue (M1) (
Figure 1. The ubiquitination pathway. (1) Ubiquitin (Ub) is linked to a cysteine residue of a Ub-activating enzyme (E1) via a reaction that uses the energy derived from the hydrolysis of an ATP molecule. (2) Ub is transferred from E1 to the cysteine of a Ub-conjugating enzyme (E2). (3) A complex is formed between E2-Ub and a Ub ligase (E3), which is able to bind a protein substrate. (4) Ub is transferred from E2 to a lysine residue of the protein substrate. Other Ub molecules can be added to the substrate-Ub complex with the same mechanism (steps 1-4). Finally, the polyubiquitinated substrate is recognized and degraded by the proteasome, whereas Ub is released and ready to start a new cycle.
Once monoubiquitination has occurred, the Ub moiety can be ubiquitinated again at one of the seven lysine sidechains (K6, K11, K27, K29, K33, K48 and K63) or at the free main-chain amine group of the N-terminal methionine residue (M1) (Figure 2), thus extending the modification into a polyubiquitin chain. Ub ability to link other Ub molecules at eight different sites is the molecular basis of a dynamic and complex system regulating cellular metabolism. In turn, the different chain types resulting from poly-ubiquitination processes dictate the specific signalling function associated with the modification [23][24][25].
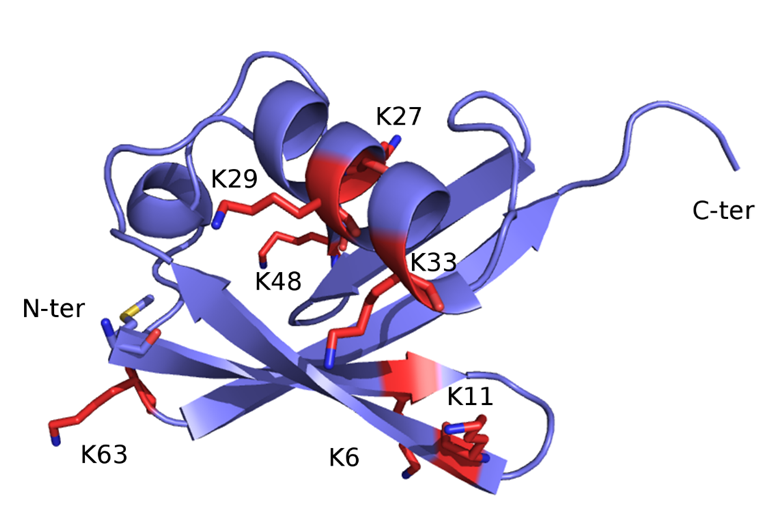
2), thus extending the modification into a polyubiquitin chain. The ability of Ub to link other Ub molecules at eight different sites is the molecular basis of a dynamic and complex system regulating cellular metabolism. In turn, the different chain types resulting from polyubiquitination processes dictate the specific signaling function associated with the modification [23,24,25].
Figure 2. X-ray structure of Ub (PDB code: 1UBQ) The structure is represented as ribbon and coloured light blue. The seven lysine residues are represented as sticks and coloured red, except for the side-chain amino group, which is blue. The N-terminal methionine residue (M1) is shown as sticks and coloured by atom-type: blue, N (of the main-chain free amino group); red, O; yellow, S; light blue, C.
Polyubiquitin linkages are classified as: i) homotypic, if Ub molecules are bound to a single lysine position of the substrate; ii) heterotypic, in case Ub molecules are bound at different lysine positions of the substrate; iii) branched, when ubiquitination takes place at two or more sites on a single Ub molecule [26]. Ub exerts several specific functions, which are contributed not only by monoubiquitin, but also by numerous different combinations of polyubiquitin linkages.
Monoubiquitination contributes to several processes, including DNA repair, control of transcription, metabolism and apoptosis[27].
The role of homotypic chains depends on the position of the lysine residue involved in the linkage. K48 Ub, where a Ub chain is covalently bonded to the ε-amino group of the lysine at position 48 of the preceding Ub molecule, is the most abundant linkage type in homotypic polyubiquitin chains, and represents the canonical signal for proteasomal degradation [28]. K11 Ub is particularly abundant as well [26] and has proteasome-independent functions, including intracellular signalling [29][30], in addition to proteasomal-dependent degradation [31]. K63 Ub regulates several processes, including the multiple translation process independent of the proteasome. This encompasses translation quality control, in particular during oxidative stress, and is also known to induce autophagy[27] [32][33].
The heterotypic branched K48 and K11 are associated with proteasomal degradation of several cell-cycle regulators, including cyclin B1 and securin, thereby promoting mitotic exit.
- The level of Huntingtin is controlled by ubiquitination.
3.1. Htt structure and function
In HD patients, the polyQ region located after the first seventeen N-terminal Htt residues is expanded beyond a threshold of 36 glutamine residues (mHtt) [34].
A distinctive feature of HD is the progressive death of striatal projection neurons (SPNs), GABAergic output neurons representing >90% of striatal cells. SPNs are divided into two groups, depending on whether they belong to the direct (DP) or indirect (IP) pathway (DP-SPNs and IP-SPNs, respectively). Both SPN sub-types receive extensive glutamatergic inputs from cortex and thalamus, and dopaminergic inputs from the ventral tegmental area and substantia nigra pars compacta [34].
Within neurons, mHtt molecules form toxic aggregates with a rate proportional to the length of the polyQ expansion. mHtt co-aggregates with a number of different proteins, decreasing their concentration in the cell. Several studies demonstrate that polyQ expansion in mHtt results in a toxic gain-of-function phenotype. Other studies, which include gene knockouts and knockdowns, demonstrate that polyQ expansion in mHtt can also have loss-of-function effects. The role of wild-type Htt should therefore be taken into account in the development of a potential therapy, as well as that of mHtt [35].
The structure of Htt in complex with HAP40 has been recently solved by cryo-EM at 4 Å resolution [19]. Conversely, unbound Htt is very difficult to study either by X-ray crystallography or cryo-EM, since it has a large size (it contains 3144 residues and weights 348 kDa), is very flexible and tends to form aggregates. Unfortunately, about 28% of Htt residues are not visible in the structure, including the first 91 residues, which comprise the 17 glutamine residues-containing polyQ expansion. In agreement with computational predictions, all secondary structure elements belonging to either Htt or HAP40 resolved in the model are α-helices, most of which (72%) are arranged in HEAT or other tandem repeats.
The cryo-EM structure revealed that Htt is formed by three domains: N-terminal and C-terminal, both of which contain multiple HEAT repeats, joined by a bridge domain (Figure 3).
The N-terminal domain (N-HEAT; residues 91-1684) forms a typical α-solenoid, comprising 21 HEAT repeats arranged in a one and a half turn right-handed superhelix. The N-HEAT was predicted to contain 2 membrane binding regions: the 1-17 N-terminal tail (not visible in the structure), which was predicted to form an amphipathic helix [36], and a larger region (comprising a.a. 168-366), containing a functionally important palmitoylation site at Cys208 [37]. This region (N-HEAT repeats 2-4) is placed on the N-HEAT convex surface and is positively charged.
The C-HEAT (a.a. 2092-3098) comprises 12 HEAT repeats that form an elliptical ring of ~80x30 Å.
The N-HEAT and C-HEAT are joined by the bridge domain. This contains 6 tandem α-helical repeats, of which repeats 3, 4 and 6 are Armadillo-like. The repeat region is flanked by 5 non-repeat helices and a flexible C-terminus (aa 2062-2092), which is unresolved.
The lack of interactions between the N-HEAT and C-HEAT domains explains the flexibility of the Htt structure in the absence of interactors. HAP40 binds to a large cleft between these two domains. Within the complex, Htt and HAP40 share large interfaces that comprise mostly hydrophobic interactions. Interestingly, bioinformatic analyses provided evidences about the evolutionary conservation of these interfaces in all Htt-encoding animal species [38].
Recently, the structure of wild-type Htt in complex with HAP40 has been compared with other mHtt-HAP40 complexes differing in the length of the polyQ repeat, namely, 46QHtt-HAP40 (46 being a typical polyQ length in HD patients) and 128QHtt-HAP40 (128 being an extremely high polyQ length). Quite surprisingly, both crosslinking mass spectrometry and cryo-EM experiments revealed no major structural differences among the different complexes, indicating that the polyQ insertion does not alter the overall Htt fold [39].
Htt function has not been fully elucidated yet, but Htt has been proposed to be involved in cellular processes such as mitotic spindle orientation, autophagy and vesicle transport [40][41][42][43]. Htt has been shown to be essential in both embryonal development and adult life. In mouse, the Htt knockdown mutant dies at about day 8.5 of gestation [44]. Additionally, Htt deletion in the mouse central nervous system leads to a phenotype similar to that of HD, i.e. cellular stress, neuroinflammation, aberrant synaptic connectivity and neuronal death [45][46][47].
Htt is crucial for the energetic metabolism not only in the brain [48] but also in peripheral tissues. In Htt-null cardiomyocytes both intracellular ATP and total purine concentration in the cellular medium were reduced. This indicates that, in the heart, Htt plays an important role in both cellular energy balance and nucleotide metabolism [49].
Recent studies carried out using mouse embryonic stem cell demonstrated that Htt is necessary for mitochondrial structure and function from the earliest stages of embryogenesis, providing a molecular explanation for the early embryonic lethality of Htt knockdown [50].
SPN death in HD have been mostly ascribed to toxic ‘‘gain-of-function’’ by mHtt. However, evidences about Htt ‘‘loss-of-function’’ contribution are also available. Indeed, wild-type Htt has been shown to be neuroprotective and, therefore, able to shield neurons against mHtt toxicity [51]. Additionally, Htt deletion in IP-SPN and DP-SPN leads to a phenotype that resembles key-features of HD, supporting the hypothesis that Htt loss-of-function contributes to SPN pathology in HD [52]. Lowering of wild-type Htt expression has also been shown to affect both health and function of primary monocyte-derived macrophages from healthy human subjects, likely by different mechanisms with respect to those associated with mHtt [53].
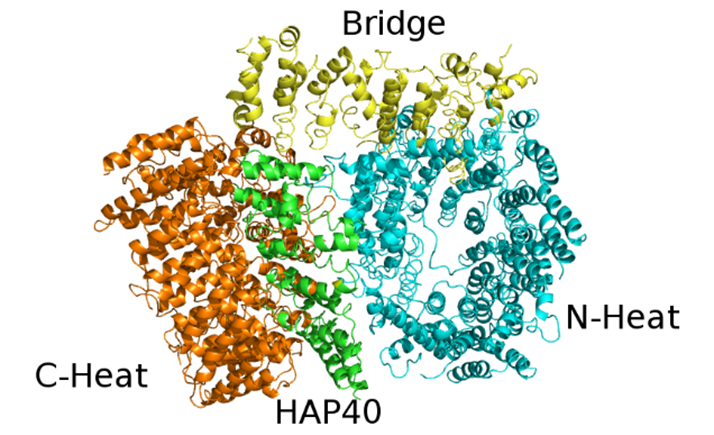
X-ray structure of Ub (PDB code: 1UBQ). The structure is represented as ribbon and colored light blue. The seven lysine residues are represented as sticks and colored red, except for the side chain amino group, which is blue. The N-terminal methionine residue (M1) is shown as sticks and colored by atom type: blue, N (of the main-chain free amino group); red, O; yellow, S; light blue, C.
Polyubiquitin linkages are classified as: (i) homotypic, if Ub molecules are bound to a single lysine position of the substrate; (ii) heterotypic, in case Ub molecules are bound at different lysine positions of the substrate; (iii) branched, when ubiquitination takes place at two or more sites on a single Ub molecule [26]. Ub exerts several specific functions, which are contributed not only by monoubiquitin, but also by numerous different combinations of polyubiquitin linkages.
Monoubiquitination contributes to several processes, including DNA repair, control of transcription, metabolism, and apoptosis [27].
The role of homotypic chains depends on the position of the lysine residue involved in the linkage. K48 Ub, wherein a Ub chain is covalently bonded to the ε-amino group of the lysine at position 48 of the preceding Ub molecule, is the most abundant linkage type in homotypic polyubiquitin chains and represents the canonical signal for proteasomal degradation [28]. K11 Ub is particularly abundant as well [26] and has proteasome-independent functions, including intracellular signaling [29,30], in addition to proteasomal-dependent degradation [31]. K63 Ub regulates several processes, including the multiple translation process independent of the proteasome. This encompasses translation quality control, in particular during oxidative stress, and is also known to induce autophagy [27,32,33].
The heterotypic, branched K48 and K11 are associated with proteasomal degradation of several cell-cycle regulators, including cyclin B1 and securin, thereby promoting mitotic exit.
3. The Level of Huntingtin Is Controlled by Ubiquitination
3.1. Htt Structure and Function
In HD patients, the polyQ region located after the first seventeen N-terminal Htt residues is expanded beyond a threshold of 36 glutamine residues (mHtt) [46].
A distinctive feature of HD is the progressive death of striatal projection neurons (SPNs), which are GABAergic output neurons representing >90% of striatal cells. SPNs are divided into two groups, depending on whether they belong to the direct (DP) or indirect (IP) pathway (DP-SPNs and IP-SPNs, respectively). Both SPN sub-types receive extensive glutamatergic inputs from cortex and thalamus, and dopaminergic inputs from the ventral tegmental area and substantia nigra pars compacta [46].
Within neurons, mHtt molecules form toxic aggregates with a rate proportional to the length of the polyQ expansion. mHtt co-aggregates with a number of different proteins, which decreases their concentration in the cell. Several studies demonstrate that polyQ expansion in mHtt results in a toxic gain-of-function phenotype. Other studies, which include gene knockouts and knockdowns, demonstrate that polyQ expansion in mHtt can also have loss-of-function effects. The role of wild-type Htt should therefore be taken into account in the development of a potential therapy, as well as that of mHtt [47].
The structure of Htt in complex with HAP40 has been recently solved by cryo-EM at 4 Å resolution [17]. Conversely, unbound Htt is very difficult to study either by X-ray crystallography or cryo-EM because it has a large size (it contains 3144 residues and weights 348 kDa), is very flexible, and tends to form aggregates. However, about 28% of Htt residues are not visible in the structure, including the first 91 residues, which comprise the 17 glutamine residue-containing polyQ expansion. In agreement with computational predictions, all secondary structure elements belonging to either Htt or HAP40 resolved in the model are α-helices, most of which (72%) are arranged in HEAT or other tandem repeats.
The cryo-EM structure revealed that Htt is formed by three domains: N-terminal domain and C-terminal domain, both of which contain multiple HEAT repeats, joined to a bridge domain (Figure 4). Figure 3. X-ray structure of the complex between HAP40 and Htt (PDB code: 6EZ8). HAP40 is coloured green and the C-Heat, Bridge and N-Heat domains of Htt are coloured orange, yellow and cyan, respectively.
3.2. Htt ubiquitination and SUMOylation
Htt is a protein with high ubiquitination potential. It contains 124 lysine residues, many of which are placed on the protein surface, as shown by cryo-EM structure analysis, and may, in principle, be ubiquitinated, or linked to other Ub-like (Ubl) proteins, such as SUMO (Small Ub-like Modifier).
Ubiquitination and/or SUMOylation has been demonstrated to take place at the 30 Htt lysine residues reported in Table 1. Ubiquitination and SUMOylation processes have been shown to compete for lysine residues K6, K9 and K15, all of which are placed within the N-terminal tail that is not visible in the solved cryo-EM structure [54], and may compete for other lysine residues as well.
4. X-ray structure of the complex between HAP40 and Htt (PDB code: 6EZ8). HAP40 is colored green and the C-Heat, Bridge, and N-Heat domains of Htt are colored orange, yellow, and cyan, respectively.
The N-terminal domain (N-HEAT; residues 91–1684) forms a typical α-solenoid, comprising 21 HEAT repeats arranged as a one-and-a-half turn, right-handed superhelix. The N-HEAT was predicted to contain two membrane binding regions: the 1–17 N-terminal tail (not visible in the structure), which was predicted to form an amphipathic helix [48], and a larger region (comprising a.a. 168–366), containing a functionally important palmitoylation site at Cys208 [49]. This region (N-HEAT repeats 2–4) is placed on the N-HEAT convex surface and is positively charged.
The C-HEAT (a.a. 2092–3098) comprises 12 HEAT repeats that form an elliptical ring of ~80 × 30 Å.
The N-HEAT and C-HEAT are joined by the bridge domain. This contains six tandem α-helical repeats, of which repeats three, four and six are Armadillo-like. The repeat region is flanked by five non-repeat helices and a flexible C-terminus (a.a. 2062–2092), which is unresolved.
The lack of interactions between the N-HEAT and C-HEAT domains explains the flexibility of the Htt structure in the absence of interactors. HAP40 binds to a large cleft between these two domains. Within the complex, Htt and HAP40 share large interfaces that comprise mostly hydrophobic interactions. Interestingly, bioinformatic analyses has provided evidence of the evolutionary conservation of these interfaces in all Htt-encoding animal species [50].
Recently, the structure of wild-type Htt in complex with HAP40 has been compared with other mHtt–HAP40 complexes differing in the length of the polyQ repeat, namely, 46QHtt–HAP40 (46 being a typical polyQ length in HD patients) and 128QHtt–HAP40 (128 being an extremely high polyQ length). Quite surprisingly, both crosslinking mass spectrometry and cryo-EM experiments revealed no major structural differences among the different complexes, indicating that the polyQ insertion does not alter the Htt fold [51].
Htt function has not yet been fully elucidated, but Htt has been proposed to be involved in cellular processes such as mitotic spindle orientation, autophagy, and vesicle transport [52,53,54,55]. Htt has been shown to be essential in both embryonal development and adult life. In mice, the Htt knockdown mutant dies at about day 8.5 of gestation [56]. Additionally, Htt deletion in the mouse central nervous system leads to a phenotype similar to that of HD, i.e., cellular stress, neuroinflammation, aberrant synaptic connectivity, and neuronal death [57,58,59].
Htt is crucial for energetic metabolism not only in the brain [60] but also in peripheral tissues. In Htt-null cardiomyocytes, both intracellular ATP and total purine concentration in the cellular medium were reduced. This indicates that, in the heart, Htt plays an important role in both cellular energy balance and nucleotide metabolism [61].
Recent studies carried out using mouse embryonic stem cell demonstrated that Htt is necessary for mitochondrial structure and function from the earliest stages of embryogenesis, providing a molecular explanation for the early embryonic lethality of Htt knockdown [62].
SPN death in HD has mostly been ascribed to toxic ‘gain-of-function’ by mHtt. However, evidence of Htt ‘loss-of-function’ contribution is also available. Indeed, wild-type Htt has been shown to be neuroprotective and, therefore, able to shield neurons against mHtt toxicity [63]. Additionally, Htt deletion in IP-SPN and DP-SPN leads to a phenotype that resembles the key features of HD, supporting the hypothesis that Htt loss-of-function contributes to SPN pathology in HD [64]. Lowering of wild-type Htt expression has also been shown to affect both health and function of primary monocyte-derived macrophages from healthy human subjects, likely by different mechanisms with respect to those associated with mHtt [65].
3.2. Htt Ubiquitination and SUMOylation
Htt is a protein with high ubiquitination potential. It contains 124 lysine residues, many of which are placed on the protein surface, as shown by cryo-EM structural analysis, and may in principle be ubiquitinated or linked to other Ub-like (Ubl) proteins, such as SUMO (Small Ub-like Modifier).
Ubiquitination and/or SUMOylation has been demonstrated for the 30 Htt lysine residues reported in Table 1. Ubiquitination and SUMOylation processes have been shown to compete for lysine residues K6, K9, and K15, all of which are placed in the N-terminal tail that is not visible in the solved cryo-EM structure [66], and they may compete for other lysine residues as well. Table 1. List of ubiquitinated and sumoylated residues in human Htt, according to PhosphoSitePlus (phosphosite.org)
. List of ubiquitinated and SUMOylated residues in human Htt, according to PhosphoSitePlus (phosphosite.org).
Residue (Human Htt) |
Demonstrated Modification |
Reference |
Lys 6 |
SUMOylation |
[66] |
Lys 9 |
SUMOylation |
[66] |
Lys 253 |
Ubiquitination |
[67] |
Lys 335 |
Ubiquitination |
[68] |
Lys 442 |
Ubiquitination |
[69] |
Lys 662 |
Ubiquitination |
[68] |
Lys 667 |
Ubiquitination |
[67] |
Lys 698 |
Ubiquitination |
[67] |
Lys 813 |
Ubiquitination |
[67] |
Lys 902 |
Ubiquitination |
[67] |
Lys 937 |
Ubiquitination |
[67] |
Lys 941 |
Ubiquitination |
[70] |
Lys 1121 |
Ubiquitination |
[68] |
Lys 1223 |
Ubiquitination |
[68] |
[ | 67 | ] |
Lys 2967 |
Ubiquitination |
[67] |
Residue (human Htt)
|
Demonstrated Modification
|
Reference
|
Lys 6
|
Sumoylation
|
[54]
|
Lys 9
|
Sumoylation
|
[54]
|
Lys 253
|
Ubiquitination
|
[55]
|
Lys 335
|
Ubiquitination
|
[56]
|
Lys 442
|
Ubiquitination
|
[57]
|
Lys 662
|
Ubiquitination
|
[56]
|
Lys 667
|
Ubiquitination
|
[55]
|
Lys 698
|
Ubiquitination
|
[55]
|
Lys 813
|
Ubiquitination
|
Lys 1431
|
Ubiquitination
|
[56], [59], [61]
|
Lys 1885
|
Ubiquitination
|
[55]
|
Lys 2417
|
Ubiquitination
|
[58]
|
Lys 2423
|
Ubiquitination
|
[56]
|
Lys 2443
|
Ubiquitination
|
[56]
|
Lys 2537
|
Ubiquitination
|
[55]
|
Lys 2564
|
Ubiquitination
|
[55]
|
Lys 2757
|
Ubiquitination
|
[55]
|
Lys 2901
|
Ubiquitination
|
[55]
|
Lys 2967
|
Ubiquitination
|
[55]
|
Ubiquitination has been linked to reduced mHtt toxicity, most likely due to increased mHtt clearance by the proteasome [62]. Conversely, SUMOylation has been shown to stabilize mHtt, reduce mHtt aggregation, enhance transcriptional dysregulation by mHtt and increase mHtt toxicity in a Drosophila model [54]. SUMOylation contribution to mHtt toxicity may be mediated by mHtt targeting to the nucleus, and hampering of ubiquitination and subsequent degradation. While Htt ubiquitination is mediated by different E3 ligases, mHtt SUMOylation is mediated by the RHES (Ras homolog enriched in striatum) protein only [63]. RHES has higher affinity for mHtt than wild-type Htt, and its selective expression in the striatum strongly suggests that this protein contributes to the HD pathology [64]. For these reasons, RHES has been considered to be an attractive target for HD therapy [65].
As described above, the presence of seven lysine residues allows Ub to transmit different signals on target proteins. Therefore, Htt polyubiquitination can take place with different mechanisms, which have not been completely understood yet. Two among the 40 E2 enzymes present in the human genome have been demonstrated to interact with Htt: UBE2W, which is known to target the N-termini of disordered proteins like tau, RBPB8 and ATXN3, as well as mHtt [66]; and UBE2K, also known as Htt interacting protein (Hip2) or E2-25k, which is a Ub-conjugating enzyme that interacts directly with Htt and may mediate its ubiquitination [67].
A variety of E3 Ub ligases have been shown to act on polyQ-containing proteins like mHtt, thereby determining a reduction of mHtt cellular levels, aggregation and cellular toxicity. Some of these E3 Ub ligases are able to poly-ubiquitinate Htt directly via K48 of Htt linked ubiquitin, allowing its clearance by the UPS. These include UBE3A/E6AP [68][69], CHIP [70], HRD1 [71], Parkin [72] and the SCF complex [73]. Other E3 ligases, like HACE1, counteract the negative effect of mHtt on redox metabolism [74]. Further E3-ligases, such as WWP1 [75] and TRAF6 [76], which are upregulated in postmortem brains of people with HD, mediate atypical Htt ubiquitination (at K6, K27 and K29), thereby inhibiting mHtt degradation and favouring the formation of ubiquitinated aggregates.
HD has been associated with global changes in the UPS, such as accumulation of K48- K63- and K11-linked poly-Ub chains, and inhibition of proteasome activity by mHtt has been proposed to contribute to neurotoxicity, although the molecular basis of this process has not been elucidated yet. The autophagic pathway has been involved in Htt aggregates clearance, and autophagy upregulation has been shown to reduce polyQ expansions toxicity in HD mouse models [77].
Only a few deubiquitinating enzymes (DUBs) have been reported to deubiquitinate Htt. ATXN3, which contains a polyQ region itself, and USP19, are the only DUBs known to target Htt besides proteasome-associated DUBs [78][79].
References
Lys 1244 |
Ubiquitination |
[ |
68 |
] |
Lys 1262 |
Ubiquitination |
[ | 71 | ] |
Lys 1337 |
Ubiquitination |
[67] |
Lys 1402 |
Ubiquitination |
[67,68,70,71,72] |
Lys 1410 |
Ubiquitination |
[67,68,70] |
Lys 1415 |
Ubiquitination |
[68] |
Lys 1431 |
Ubiquitination |
[68,70,73] |
Lys 1885 |
Ubiquitination |
[67] |
Lys 2417 |
Ubiquitination |
[71] |
Lys 2423 |
Ubiquitination |
[68] |
Lys 2443 |
Ubiquitination |
[68] |
Lys 2537 |
Ubiquitination |
[67] |
Lys 2564 |
Ubiquitination |
[67] |
Lys 2757 |
Ubiquitination |
[67] |
Lys 2901 |
Ubiquitination |
|
[55] |
|
|
Lys 902 |
|
Ubiquitination
|
[55]
|
Lys 937
|
Ubiquitination
|
[55]
|
Lys 941
|
Ubiquitination
|
[56]
|
Lys 1121
|
Ubiquitination
|
[56]
|
Lys 1223
|
Ubiquitination
|
[56]
|
Lys 1244
|
Ubiquitination
|
[56]
|
Lys 1262
|
Ubiquitination
|
[58]
|
Lys 1337
|
Ubiquitination
|
[55]
|
Lys 1402
|
Ubiquitination
|
[55],[56], [58],[59], [60]
|
Lys 1410
|
Ubiquitination
|
[55], [56], [59]
|
Lys 1415
|
Ubiquitination
|
[56]
|
- Fisher ER, Hayden MR. Multisource ascertainment of Huntington disease in Canada: prevalence and population at risk. Mov Disord [Internet]. 2014 Jan;29(1):105–14.
- Kay C, Hayden MR, Leavitt BR. Epidemiology of Huntington disease. Handb Clin Neurol [Internet]. 2017;144:31–46.
- Crowell V, Houghton R, Tomar A, Fernandes T, Squitieri F. Modeling Manifest Huntington’s Disease Prevalence Using Diagnosed Incidence and Survival Time. Neuroepidemiology. 2021;55(5):361–8.
- Toczek M, Zielonka D, Zukowska P, Marcinkowski JT, Slominska E, Isalan M, et al. An impaired metabolism of nucleotides underpins a novel mechanism of cardiac remodeling leading to Huntington’s disease related cardiomyopathy. Biochim Biophys Acta [Internet]. 2016 Nov;1862(11):2147–57.
- Mielcarek M, Smolenski RT, Isalan M. Transcriptional Signature of an Altered Purine Metabolism in the Skeletal Muscle of a Huntington’s Disease Mouse Model. Front Physiol. 2017;8:127.
- Mielcarek M. Huntington’s disease is a multi-system disorder. Rare Dis (Austin, Tex) [Internet]. 2015;3(1):e1058464.
- Kremer B, Goldberg P, Andrew SE, Theilmann J, Telenius H, Zeisler J, et al. A worldwide study of the Huntington’s disease mutation. The sensitivity and specificity of measuring CAG repeats. N Engl J Med [Internet]. 1994 May;330(20):1401–6.
- DiFiglia M, Sapp E, Chase KO, Davies SW, Bates GP, Vonsattel JP, et al. Aggregation of huntingtin in neuronal intranuclear inclusions and dystrophic neurites in brain. Science (80- ) [Internet]. 1997;277(5334):1990–3.
- Gutekunst CA, Li SH, Yi H, Mulroy JS, Kuemmerle S, Jones R, et al. Nuclear and neuropil aggregates in Huntington’s disease: Relationship to neuropathology. J Neurosci [Internet]. 1999;19(7):2522–34.
- Quarrell O, O’Donovan KL, Bandmann O, Strong M. The Prevalence of Juvenile Huntington’s Disease: A Review of the Literature and Meta-Analysis. PLoS Curr [Internet]. 2012 Jul;4:e4f8606b742ef3.
- Fusilli C, Migliore S, Mazza T, Consoli F, De Luca A, Barbagallo G, et al. Biological and clinical manifestations of juvenile Huntington’s disease: a retrospective analysis. Lancet Neurol. 2018;17(11):986–93.
- Tydlacka S, Wang CE, Wang X, Li S, Li XJ. Differential activities of the ubiquitin-proteasome system in neurons versus glia may account for the preferential accumulation of misfolded proteins in neurons. J Neurosci [Internet]. 2008;28(49):13285–95.
- Li XJ, Li H, Li S. Clearance of mutant huntingtin. Autophagy [Internet]. 2010;6(5):663–4.
- Li X, Wang CE, Huang S, Xu X, Li XJ, Li H, et al. Inhibiting the ubiquitin-proteasome system leads to preferential accumulation of toxic N-terminal mutant huntingtin fragments. Hum Mol Genet [Internet]. 2010;19(12):2445–55.
- Pratt WB, Gestwicki JE, Osawa Y, Lieberman AP. Targeting Proteostasis Through the Protein Quality Control Function of the Hsp90/Hsp70-based Chaperone Machinery for Treatment of Adult Onset Neurodegenerative Diseases. Annu Rev Pharmacol Toxicol. 2015;55:353–71.
- Lackie RE, Maciejewski A, Ostapchenko VG, Marques-Lopes J, Choy W-Y, Duennwald ML, et al. The Hsp70/Hsp90 Chaperone Machinery in Neurodegenerative Diseases. Front Neurosci. 2017;11:254.
- Qi L, Zhang X-D, Wu J-C, Lin F, Wang J, DiFiglia M, et al. The Role of Chaperone-Mediated Autophagy in Huntingtin Degradation. PLoS One. 2012;7(10):1–16.
- Baldo B, Weiss A, Parker CN, Bibel M, Paganetti P, Kaupmann K. A screen for enhancers of clearance identifies huntingtin as a heat shock protein 90 (Hsp90) client protein. J Biol Chem [Internet]. 2012;287(2):1406–14.
- Guo Q, Huang B, Cheng J, Seefelder M, Engler T, Pfeifer G, et al. The cryo-electron microscopy structure of huntingtin. Nature [Internet]. 2018 Mar;555(7694):117–20.
- Hershko A, Ciechanover A. The ubiquitin system. Annu Rev Biochem [Internet]. 1998;67:425–79.
- Ciechanover A, Elias S, Heller H, Hershko A. “Covalent affinity” purification of ubiquitin-activating enzyme. J Biol Chem [Internet]. 1982 Mar;257(5):2537–42.
- Hershko A, Heller H. Occurrence of a polyubiquitin structure in ubiquitin-protein conjugates. Biochem Biophys Res Commun [Internet]. 1985 May;128(3):1079–86.
- Komander D, Rape M. The ubiquitin code. Annu Rev Biochem [Internet]. 2012;81:203–29.
- Ye Y, Blaser G, Horrocks MH, Ruedas-Rama MJ, Ibrahim S, Zhukov AA, et al. Ubiquitin chain conformation regulates recognition and activity of interacting proteins. Nature [Internet]. 2012 Dec;492(7428):266–70.
- Swatek KN, Komander D. Ubiquitin modifications. Cell Res [Internet]. 2016 Apr;26(4):399–422.
- Grice GL, Lobb IT, Weekes MP, Gygi SP, Antrobus R, Nathan JA. The Proteasome Distinguishes between Heterotypic and Homotypic Lysine-11-Linked Polyubiquitin Chains. Cell Rep [Internet]. 2015 Jul;12(4):545–53.
- Dougherty SE, Maduka AO, Inada T, Silva GM. Expanding Role of Ubiquitin in Translational Control. Int J Mol Sci. 2020;21(3):1–24.
- Ohtake F, Tsuchiya H. The emerging complexity of ubiquitin architecture. J Biochem [Internet]. 2017 Feb;161(2):125–33.
- Bremm A, Freund SM V, Komander D. Lys11-linked ubiquitin chains adopt compact conformations and are preferentially hydrolyzed by the deubiquitinase Cezanne. Nat Struct Mol Biol [Internet]. 2010 Aug;17(8):939–47.
- Dynek JN, Goncharov T, Dueber EC, Fedorova A V, Izrael-Tomasevic A, Phu L, et al. c-IAP1 and UbcH5 promote K11-linked polyubiquitination of RIP1 in TNF signalling. EMBO J [Internet]. 2010 Dec;29(24):4198–209.
- Min M, Mevissen TET, De Luca M, Komander D, Lindon C. Effcient APC/C substrate degradation in cells undergoing mitotic exit depends on K11 ubiquitin linkages. Mol Biol Cell [Internet]. 2015;26(24):4325–32.
- Saito K, Horikawa W, Ito K. Inhibiting K63 Polyubiquitination Abolishes No-Go Type Stalled Translation Surveillance in Saccharomyces cerevisiae. PLoS Genet [Internet]. 2015;11(4):1–18.
- Silva GM, Finley D, Vogel C. K63 polyubiquitination is a new modulator of the oxidative stress response. Nat Struct Mol Biol [Internet]. 2015 Feb;22(2):116–23.
- Vonsattel JP, Myers RH, Stevens TJ, Ferrante RJ, Bird ED, Richardson EPJ. Neuropathological classification of Huntington’s disease. J Neuropathol Exp Neurol [Internet]. 1985 Nov;44(6):559–77.
- Schulte J, Littleton JT. The biological function of the Huntingtin protein and its relevance to Huntington’s Disease pathology. Curr Trends Neurol [Internet]. 2011 Jan;5:65–78.
- Arndt JR, Chaibva M, Legleiter J. The emerging role of the first 17 amino acids of huntingtin in Huntington’s disease. Biomol Concepts [Internet]. 2015 Mar;6(1):33–46.
- Yanai A, Huang K, Kang R, Singaraja RR, Arstikaitis P, Gan L, et al. Palmitoylation of huntingtin by HIP14 is essential for its trafficking and function. Nat Neurosci [Internet]. 2006 Jun;9(6):824–31.
- Seefelder M, Alva V, Huang B, Engler T, Baumeister W, Guo Q, et al. The evolution of the huntingtin-associated protein 40 (HAP40) in conjunction with huntingtin. BMC Evol Biol [Internet]. 2020 Dec;20(1):162.
- Huang B, Guo Q, Niedermeier ML, Cheng J, Engler T, Maurer M, et al. Pathological polyQ expansion does not alter the conformation of the Huntingtin-HAP40 complex. Structure [Internet]. 2021 Apr;
- Rui Y-N, Xu Z, Patel B, Chen Z, Chen D, Tito A, et al. Huntingtin functions as a scaffold for selective macroautophagy. Nat Cell Biol [Internet]. 2015 Mar;17(3):262–75.
- Caviston JP, Ross JL, Antony SM, Tokito M, Holzbaur ELF. Huntingtin facilitates dynein/dynactin-mediated vesicle transport. Proc Natl Acad Sci U S A [Internet]. 2007 Jun;104(24):10045–50.
- Gauthier LR, Charrin BC, Borrell-Pagès M, Dompierre JP, Rangone H, Cordelières FP, et al. Huntingtin controls neurotrophic support and survival of neurons by enhancing BDNF vesicular transport along microtubules. Cell [Internet]. 2004 Jul;118(1):127–38.
- Godin JD, Colombo K, Molina-Calavita M, Keryer G, Zala D, Charrin BC, et al. Huntingtin is required for mitotic spindle orientation and mammalian neurogenesis. Neuron [Internet]. 2010 Aug;67(3):392–406.
- Zeitlin S, Liu JP, Chapman DL, Papaioannou VE, Efstratiadis A. Increased apoptosis and early embryonic lethality in mice nullizygous for the Huntington’s disease gene homologue. Nat Genet [Internet]. 1995 Oct;11(2):155–63.
- McKinstry SU, Karadeniz YB, Worthington AK, Hayrapetyan VY, Ozlu MI, Serafin-Molina K, et al. Huntingtin is required for normal excitatory synapse development in cortical and striatal circuits. J Neurosci [Internet]. 2014 Jul;34(28):9455–72.
- Dragatsis I, Levine MS, Zeitlin S. Inactivation of Hdh in the brain and testis results in progressive neurodegeneration and sterility in mice. Nat Genet [Internet]. 2000 Nov;26(3):300–6.
- Mehler MF, Petronglo JR, Arteaga-Bracho EE, Gulinello ME, Winchester ML, Pichamoorthy N, et al. Loss-of-Huntingtin in Medial and Lateral Ganglionic Lineages Differentially Disrupts Regional Interneuron and Projection Neuron Subtypes and Promotes Huntington’s Disease-Associated Behavioral, Cellular, and Pathological Hallmarks. J Neurosci [Internet]. 2019 Mar;39(10):1892–909.
- Morea V, Bidollari E, Colotti G, Fiorillo A, Rosati J, De Filippis L, et al. Glucose transportation in the brain and its impairment in Huntington disease: one more shade of the energetic metabolism failure? Amino Acids. 2017;49(7):1147–57.
- Tomczyk M, Glaser T, Ulrich H, Slominska EM, Smolenski RT. Huntingtin protein maintains balanced energetics in mouse cardiomyocytes. Nucleosides Nucleotides Nucleic Acids [Internet]. 2020 Sep;1–8.
- Ismailoglu I, Chen Q, Popowski M, Yang L, Gross SS, Brivanlou AH. Huntingtin protein is essential for mitochondrial metabolism, bioenergetics and structure in murine embryonic stem cells. Dev Biol [Internet]. 2014 Jul;391(2):230–40.
- Leavitt BR, van Raamsdonk JM, Shehadeh J, Fernandes H, Murphy Z, Graham RK, et al. Wild-type huntingtin protects neurons from excitotoxicity. J Neurochem [Internet]. 2006 Feb;96(4):1121–9.
- Burrus CJ, McKinstry SU, Kim N, Ozlu MI, Santoki A V, Fang FY, et al. Striatal Projection Neurons Require Huntingtin for Synaptic Connectivity and Survival. Cell Rep [Internet]. 2020 Jan;30(3):642-657.e6.
- O’Regan GC, Farag SH, Ostroff GR, Tabrizi SJ, Andre R. Wild-type huntingtin regulates human macrophage function. Sci Rep [Internet]. 2020 Oct;10(1):17269.
- Steffan JS, Agrawal N, Pallos J, Rockabrand E, Trotman LC, Slepko N, et al. SUMO Modification of Huntingtin and Huntington’s Disease Pathology. Science (80- ) [Internet]. 2004;304(5667):100–4.
- Udeshi ND, Svinkina T, Mertins P, Kuhn E, Mani DR, Qiao JW, et al. Refined preparation and use of anti-diglycine remnant (K-ε-GG) antibody enables routine quantification of 10,000s of ubiquitination sites in single proteomics experiments. Mol Cell Proteomics [Internet]. 2013 Mar;12(3):825–31.
- Akimov V, Barrio-Hernandez I, Hansen SVF, Hallenborg P, Pedersen A-K, Bekker-Jensen DB, et al. UbiSite approach for comprehensive mapping of lysine and N-terminal ubiquitination sites. Nat Struct Mol Biol [Internet]. 2018 Jul;25(7):631–40.
- Dong G, Callegari E, Gloeckner CJ, Ueffing M, Wang H. Mass spectrometric identification of novel posttranslational modification sites in Huntingtin. Proteomics [Internet]. 2012 Jun;12(12):2060–4.
- Wagner SA, Beli P, Weinert BT, Nielsen ML, Cox J, Mann M, et al. A proteome-wide, quantitative survey of in vivo ubiquitylation sites reveals widespread regulatory roles. Mol Cell Proteomics [Internet]. 2011 Oct;10(10):M111.013284.
- Mertins P, Qiao JW, Patel J, Udeshi ND, Clauser KR, Mani DR, et al. Integrated proteomic analysis of post-translational modifications by serial enrichment. Nat Methods [Internet]. 2013 Jul;10(7):634–7.
- Boeing S, Williamson L, Encheva V, Gori I, Saunders RE, Instrell R, et al. Multiomic Analysis of the UV-Induced DNA Damage Response. Cell Rep [Internet]. 2016 May;15(7):1597–610.
- Povlsen LK, Beli P, Wagner SA, Poulsen SL, Sylvestersen KB, Poulsen JW, et al. Systems-wide analysis of ubiquitylation dynamics reveals a key role for PAF15 ubiquitylation in DNA-damage bypass. Nat Cell Biol [Internet]. 2012 Oct;14(10):1089–98.
- Kalchman MA, Graham RK, Xia G, Koide HB, Hodgson JG, Graham KC, et al. Huntingtin is ubiquitinated and interacts with a specific ubiquitin-conjugating enzyme. J Biol Chem [Internet]. 1996 Aug;271(32):19385–94.
- Subramaniam S, Sixt KM, Barrow R, Snyder SH. Rhes, a striatal specific protein, mediates mutant-huntingtin cytotoxicity. Science. 2009;324(5932):1327–30.
- Falk JD, Vargiu P, Foye PE, Usui H, Perez J, Danielson PE, et al. Rhes: A striatal-specific Ras homolog related to Dexras1. J Neurosci Res. 1999;57(6):782–8.
- Carbo M, Brandi V, Pascarella G, Staid DS, Colotti G, Polticelli F, et al. Bioinformatics analysis of Ras homologue enriched in the striatum, a potential target for Huntington’s disease therapy. Int J Mol Med [Internet]. 2019 Dec;44(6):2223–33.
- Wang B, Zeng L, Merillat SA, Fischer S, Ochaba J, Thompson LM, et al. The ubiquitin conjugating enzyme Ube2W regulates solubility of the Huntington’s disease protein, huntingtin. Neurobiol Dis [Internet]. 2018 Jan;109(Pt A):127–36.
- de Pril R, Fischer DF, Roos RAC, van Leeuwen FW. Ubiquitin-conjugating enzyme E2-25K increases aggregate formation and cell death in polyglutamine diseases. Mol Cell Neurosci [Internet]. 2007 Jan;34(1):10–9.
- Maheshwari M, Shekhar S, Singh BK, Jamal I, Vatsa N, Kumar V, et al. Deficiency of Ube3a in Huntington’s disease mice brain increases aggregate load and accelerates disease pathology. Hum Mol Genet. 2014;23(23):6235–45.
- Mishra A, Dikshit P, Purkayastha S, Sharma J, Nukina N, Jana NR. E6-AP promotes misfolded polyglutamine proteins for proteasomal degradation and suppresses polyglutamine protein aggregation and toxicity. J Biol Chem [Internet]. 2008 Mar;283(12):7648–56.
- Joshi V, Amanullah A, Upadhyay A, Mishra R, Kumar A, Mishra A. A decade of boon or burden: What has the chip ever done for cellular protein quality control mechanism implicated in neurodegeneration and aging? Front Mol Neurosci [Internet]. 2016;9(OCT2016).
- Yang H, Zhong X, Ballar P, Luo S, Shen Y, Rubinsztein DC, et al. Ubiquitin ligase Hrd1 enhances the degradation and suppresses the toxicity of polyglutamine-expanded huntingtin. Exp Cell Res [Internet]. 2007;313(3):538–50.
- Rubio I, Rodríguez-Navarro JA, Tomás-Zapico C, Ruíz C, Casarejos MJ, Perucho J, et al. Effects of partial suppression of parkin on huntingtin mutant R6/1 mice. Brain Res. 2009;1281:91–100.
- Bhutani S, Das A, Maheshwari M, Lakhotia SC, Jana NR. Dysregulation of core components of SCF complex in poly-glutamine disorders. Cell Death Dis [Internet]. 2012;3(11):1–10.
- Rotblat B, Southwell AL, Ehrnhoefer DE, Skotte NH, Metzler M, Franciosi S, et al. HACE1 reduces oxidative stress and mutant Huntingtin toxicity by promoting the NRF2 response. Proc Natl Acad Sci U S A [Internet]. 2014;111(8):3032–7.
- Lin L, Jin Z, Tan H, Xu Q, Peng T, Li H. Atypical ubiquitination by E3 ligase WWP1 inhibits the proteasome-mediated degradation of mutant huntingtin. Brain Res [Internet]. 2016;1643:103–12.
- Zucchelli S, Marcuzzi F, Codrich M, Agostoni E, Vilotti S, Biagioli M, et al. Tumor necrosis factor receptor-associated factor 6 (TRAF6) associates with Huntingtin protein and promotes its atypical ubiquitination to enhance aggregate formation. J Biol Chem [Internet]. 2011;286(28):25108–17.
- Harding RJ, Tong Y-F. Proteostasis in Huntington’s disease: disease mechanisms and therapeutic opportunities. Acta Pharmacol Sin [Internet]. 2018 May;39(5):754–69.
- Tsou W-L, Ouyang M, Hosking RR, Sutton JR, Blount JR, Burr AA, et al. The deubiquitinase ataxin-3 requires Rad23 and DnaJ-1 for its neuroprotective role in Drosophila melanogaster. Neurobiol Dis [Internet]. 2015 Oct;82:12–21.
- He W-T, Xue W, Gao Y-G, Hong J-Y, Yue H-W, Jiang L-L, et al. HSP90 recognizes the N-terminus of huntingtin involved in regulation of huntingtin aggregation by USP19. Sci Rep [Internet]. 2017 Nov;7(1):14797.
Ubiquitination has been linked to reduced mHtt toxicity, most likely due to increased mHtt clearance by the proteasome [74]. Conversely, SUMOylation has been shown to stabilize mHtt, reduce mHtt aggregation, enhance transcriptional dysregulation by mHtt, and increase mHtt toxicity in a Drosophila model [66]. SUMOylation contribution to mHtt toxicity may be mediated by mHtt targeting of the nucleus and the hampering of ubiquitination and subsequent degradation. While Htt ubiquitination is mediated by different E3 ligases, mHtt SUMOylation is mediated by only one protein, i.e., RHES (Ras homolog enriched in striatum) [75]. RHES has higher affinity for mHtt than wild-type Htt, and its selective expression in the striatum strongly suggests that this protein contributes to the HD pathology [76]. For these reasons, RHES has been considered to be an attractive target for HD therapy [77].
As described above, the presence of seven lysine residues allows Ub to transmit different signals on target proteins. Therefore, Htt polyubiquitination can take place with different mechanisms, which have not yet been completely understood. Two among the 40 E2 enzymes present in the human genome have been demonstrated to interact with Htt: UBE2W, which is known to target the N-termini of disordered proteins such as Tau, RBPB8, and ATXN3, as well as mHtt [78]; and UBE2K, also known as Htt-interacting protein (Hip2) or E2-25k, which is a Ub-conjugating enzyme that directly interacts with Htt and may mediate its ubiquitination [79].