Drug targeting and nanomedicine are different strategies for improving the delivery of drugs to their target. Several antibodies, immuno-drug conjugates and nanomedicines are already approved and used in clinics, demonstrating the potential of such approaches, including the recent examples of the DNA- and RNA-based vaccines against COVID-19 infections. Nevertheless, targeting remains a major challenge in drug delivery and different aspects of how these objects are processed at organism and cell level still remain unclear, hampering the further development of efficient targeted drugs.
1. Introduction
Nanosized drug carriers (nanomedicines) and smaller drug targeting constructs are used in the field of advanced drug delivery systems to improve the delivery of drugs to the target site. They can achieve this via different mechanisms and, if combined, these two strategies are an extremely powerful tool for future innovative therapies. Nanomedicines are typically larger constructs of variable size (between 10–500 nm) and composition (polymers, lipids or inorganic components, for instance, gold or silica) that can accommodate large quantities of therapeutic entities
[1][2][3][1,2,3]. Drug delivery to the desired site of action is favored by a prolonged circulation time in blood, which is achieved via different mechanisms, such as for instance by the prevention of renal clearance through size increase and by protecting the therapeutic compounds from degradation in plasma through shielding. A prolonged circulation time favors the accumulation in the target area. In the case of tumor targeting, this is often achieved by the so-called enhanced permeability and retention (EPR) effect, where the nanomedicine passively accumulates into the tumor tissue by diffusion through leaky tumor blood vessels
[4][5][4,5]. Similar EPR effects can be exploited also to target inflamed areas and other diseases characterized by leaky blood vessels
[6]. Recent findings however have challenged
the grou
p'sr current understanding on the mechanisms of nanoparticle accumulation into tumors and suggested that this mainly occurs via specific active uptake mechanisms into tumor endothelial cells and later transcytosis into the tumor tissue
[6][7][8][6,7,8]. At the same time, nanoparticles may also adsorb plasma components that may induce accumulation in the target area. Pegylated liposomal doxorubicin (Doxil)
[7][9][7,9] is an example of so-called passively targeted drug exploiting the EPR effect and one of the first nanomedicines approved for clinical use (1995), while Onpattro
[10][11][12][10,11,12] that adsorbs apolipoprotein E (apoE) in plasma and is then taken up by the apoE or low density lipoprotein receptor (LDLR))
[13] on hepatocytes is an example of the latter strategy and was recently approved for clinical use (2018). More examples show the applicability of this approach
[14].
Smaller drug targeting constructs, which in some definitions are also included among nanomedicines, instead make use of active targeting ligands to induce drug uptake in the target area or target cells. Such targeting ligands bind to a specific receptor on designated target cells. Obviously, nanomedicines using a combination of passive and active targeting are also highly investigated, although their success so far has been limited and has also been highly debated
[15][16][17][15,16,17]. Active targeting approaches are mostly antibody-mediated targeting strategies but can also comprise the coupling of other targeting ligands such as lipoproteins, peptides, sugar moieties, aptamers and smaller epitopes.
The development of different nanoparticles as drug carriers has gained speed in recent years since Doxil approval, but the application of this concept in patients is still hampered by serious hurdles like for instance uptake by the reticulo-endothelial system
[18] and corona formation that affect biodistribution and efficacy
[19][20][21][19,20,21]. Moreover, the development of actively targeted drugs has seen tremendous growth in recent years, but most targets, that is, pathogenic cells that play a key role in disease progression, can still not be reached selectively by carriers.
Overall, both larger drug delivery systems and smaller targeted constructs constitute versatile platforms for multiple therapeutic entities. The requirements of such platforms may differ because both types of therapeutics are subjected to different clearance mechanisms and driving forces that affect their distribution in plasma, tissues and within cells. In all cases, the first step for the development of efficient drug delivery systems and targeted drugs is to obtain fundamental knowledge on these mechanisms and the existing driving forces.
2. Nanomedicines to Delivery Drugs into Target Cells
In comparison to the simpler targeted drug constructs, larger nano-sized materials (typically in the range of tens to few hundreds nanometer) can be loaded with higher amounts of drugs and be used as a drug carrier to improve the delivery to the target cells. Nanomedicines can be made from multiple types of materials, including lipid-based and polymer-based nanomedicines, bio-inspired materials (including for instance viral vectors and natural nanoparticles such as exosomes and lipid nanoparticles), and inorganic nanoparticles
[22][132].
Figure 12 shows a simplified scheme of a nanomedicine. Active targeting can be coupled to the drug carrier by decorating the nanomedicine with targeting ligands.
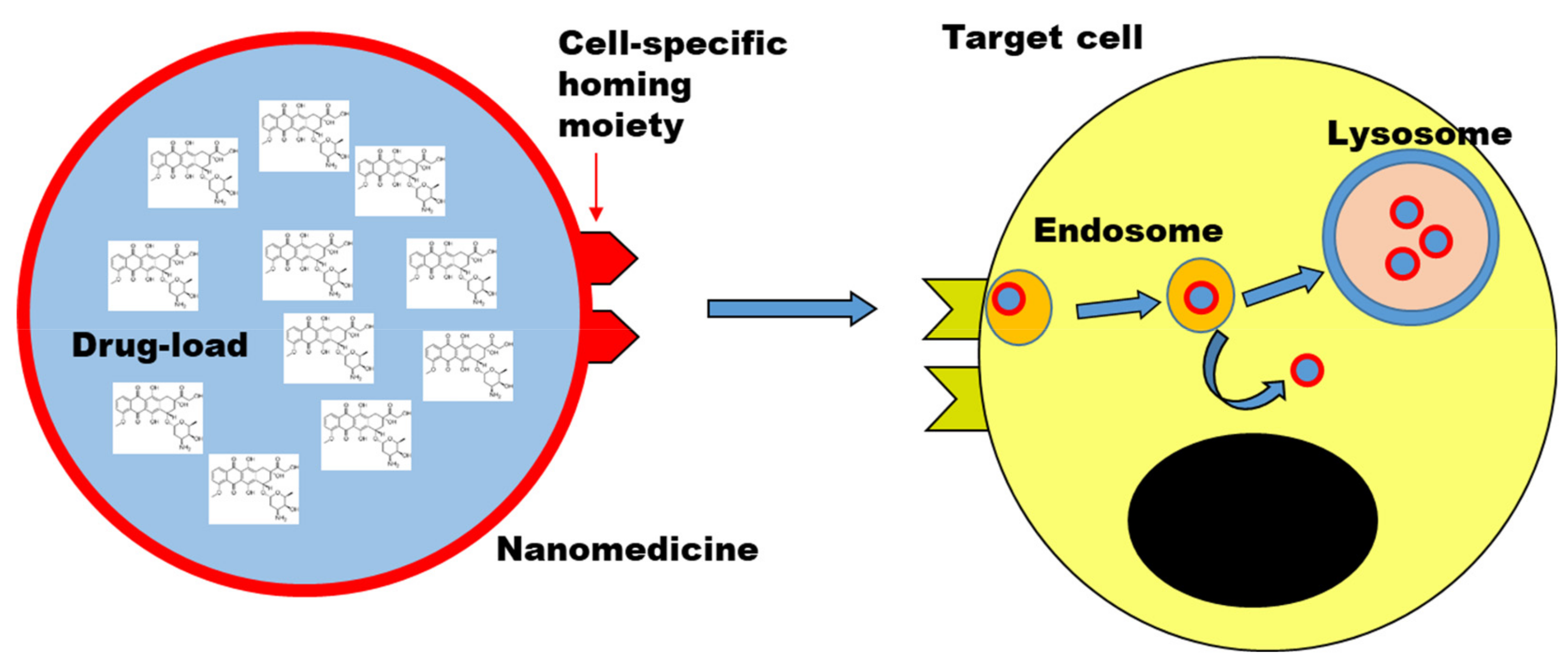
Figure 12. Nanomedicine to deliver drugs to target cells. Scheme illustrating a nanomedicine carrying a high-load of drug molecules to a target cell. Drug targeting strategies can be combined by decorating the nanomedicine with targeting ligands (cell-specific homing moiety). Upon uptake by cells, typically by endocytosis, nanomedicines are trafficked along the endo-lysosomal pathway, unless designed to avoid it (i.e., endosomal escape strategies). Objects not to scale.
Although quite different in their structure, several important features are common between smaller targeted constructs and these larger drug carriers, and the way they are processed at tissue and cell level. A key aspect in comparison to small molecular drug compounds is that these advanced drug delivery systems are too large to simply diffuse into cells, rather they are internalized by cells via active mechanisms of endocytosis which require cell energy, and are then processed by cells into specific locations (usually the lysosomes, see later for further details), instead of partitioning based on their solubility
[23][24][133,134]. This is also one of the reason why sometimes they are both classified as nanomedicines, in order to stress the common aspects in the way they are processed because of their (larger) nano-scale size.
In order to achieve efficient targeting and drug delivery, a better understanding of how these objects are processed at organism and at the cell level is required, so that their design can be changed in order to control their behavior and efficacy.
We discuss in the next sections some key aspects that need to be understood and controlled towards this aim.
3. Controlling the Interactions of Targeted Drugs and Nanomedicines with Cells
3.1. Interactions with Biological Fluids
Overall, the examples summarized in
Table 12 highlight that a better understanding of the complex interactions of nanomedicine in biological fluids and the effects of corona formation on distribution and uptake by cells is necessary for the design of successful targeted drugs and nanomedicines. At the same time such knowledge can help to discover novel strategies for targeting and to prolong nanomedicine plasma residence time.
Table 12. Examples of effects mediated by corona biomolecules adsorbed on nanoparticles and other nanoparticle modifications affecting corona formation. The Table summarizes some examples of the effects mediated by the corona forming on nanoparticles which have been described in literature
. and which Hereare discussed in
Section 6.1. We stress that the Table is not complete and just includes some selected examples as a reference.
Nanoparticle Modification or Corona Component |
Effect Reported |
Selected Examples |
Corona formation |
Can mask targeting ligands in vitro |
[25][139] |
Opsonin proteins in the corona |
Activation of immune cells, nanoparticle removal from circulation |
[26][27][28][135,136,137] |
PEGylation |
Reduced protein adsorption and/or binding of dysopsonin proteins in the corona such as clusterin |
[26][29][30][135,141,152] |
Dysopsonin proteins in the corona |
Prolonged circulation time |
[31][150] |
Albumin in the corona |
Prolonged circulation time |
[32][151] |
Clusterin (apolipoprotein J) in the corona |
Prolonged circulation time |
[30][152] |
Histidine rich glycoprotein in the corona |
Prolonged circulation time |
[33][34][153,154] |
CD47 functionalization |
Marker of self, “don’t eat me” signal for immune cells, prolonged circulation |
[35][143] |
Leukocytes cell membrane coating |
Nanoparticle camouflage, prolonged circulation and increased accumulation in inflamed areas |
[36][142] |
Red-cell membrane coating |
Nanoparticle camouflage, prolonged circulation |
[37][144] |
Apolipoprotein B in the corona |
Uptake mediated by LDLR |
[38][39][148,149] |
Apolipoprotein E in the corona |
In vivo targeting of liver hepatocytes via LDLR |
[11] |
Apolipoprotein E in the corona |
Promotes nanoparticle transcytosis across the blood brain barrier |
[40][147] |
Vitronectin in the corona |
Increased uptake via ανβ3 integrin receptor in vitro and in vivo |
[41][42][145,146] |
3.2. Interactions with Cells and Intracellular Fate
After administration and modifications in biological fluids, the next steps into the journey of nanomedicines and targeted drugs are the interactions at the cell membrane both of the targeted cells and all other cells, and the following uptake mechanism and intracellular trafficking.
While
hwe
rein discussed above examples of drug targeting strategies and effects of corona formation on targeting and interactions with cell receptors, there are other key aspects in the interactions at the cell membrane of nanomedicines and targeted drugs which need to be better understood and controlled in order to improve their efficacy.
One important aspect that has emerged is that even when specific receptors are targeted (via active targeting ligands or via the corona adsorbing on nanomedicines), the targeted drug or nanomedicine may be internalized and processed by cells in different ways comparing to what usually observed for the same receptors and their natural ligands
[43][161]. For instance,
itwe is found that even when the LDLR mediates nanoparticle uptake, the mechanisms of internalization is not clathrin mediated, as usually observed for this receptor
[38][148]. Similarly, nanoparticles targeting the transferrin receptor were not recycled and exported by cells as transferrin, but were trafficked by cells towards the lysosomes
[25][139]. More efforts are needed to understand how after interactions with certain receptors, cells internalize and process these materials. Without this knowledge, the development of nanomedicines and targeted drugs risks to remain mainly limited to screening of multiple materials, as opposed to the tailored design of materials with the properties required to achieve the desired outcomes at cell level (as well as in vivo).
The mechanisms cells use for the internalization of nanomedicine and targeted constructs also affect uptake efficiency and intracellular trafficking kinetics
[24][43][134,161]. These factors affect the time required to reach the therapeutic dose intracellularly, and the time required to reach specific locations inside cells, which all together contribute to the final therapeutic efficacy.
Characterizing the mechanisms by which nanomedicines and targeted drugs are internalized by cells remains highly challenging
[24][43][44][134,161,162]. Many contrasting results are often reported and it is hard to draw conclusions on how nanoparticle properties affect the mechanism of uptake or how they vary among different cell types. This is also due to complicating factors such as corona formation, which are not always controlled and reproduced among different studies. Additionally, the methods usually applied to try to characterize these mechanisms are often limited
[24][44][134,162].
HWe
rein have shown examples of this for classic transport inhibitors commonly used for this purpose
[45][163]. For instance
itwe is found that when using high concentration of inhibitors such as chlorpromazine, a common drug to block clathrin-mediated endocytosis, reduced nanoparticle uptake was observed but this was due to strong toxicity on cells, as opposed to a specific inhibition of the pathway. Additionally, many of these compounds lost their efficacy when applied to cells in a medium with serum, probably due to protein binding
[45][163]. Given the effect of corona formation on targeting and nanomedicine interactions with cells, thus the need to include serum when testing nanomedicine and targeted drugs in vitro, other compounds whose efficacy is not affected by the presence of serum need to be used
[43][45][161,163]. Thus, without controls for similar effects and to exclude toxicity, results obtained with these common transport inhibitors may lead to wrong conclusions. Given these limits, multiple methods need to be combined. Furthermore,
itwe cannot
be exclude
d that nano-sized materials may be processed in different ways than natural ligands and alternative endocytic mechanisms not yet characterized may be triggered by these special cargoes. Thus, new methods need to be developed to identify potential novel targets not yet associated to cell uptake mechanisms. To this aim,
hereinwe have applied genome-wide screening and developed proteomic based approaches to identify all genes and proteins involved in nanoparticle uptake (Montizaan et al., unpublished; Garcia-Romeu et al., unpublished). Indeed, many novel targets have been identified thanks to this kind of approaches and current efforts are focused on understanding their role.
Finally, it is commonly observed that after uptake most nanomedicines are trafficked by cells along the endo-lysosomal pathway towards the lysosomes
[23][46][133,164]. While this can be exploited when these are the targeted intracellular compartments, all drugs which require accumulation into other locations inside cells require to escape the endo-lysosomal compartments or are otherwise degraded once they are transported in the lysosomes. Several strategies for endosomal escape have been investigated, and often are limited by toxicity and poor efficacy
[47][48][165,166]. In the case of RNA delivery and recently approved lipid nanoparticles such as Onpattro or the RNA vaccines against COVID-19, this has been achieved utilizing ionizable lipids which become positively charged only once inside the slightly acidic environment of the endosome
[11][12][11,12]. The acquired cationic charge can destabilize the endosomal membrane and promote escape of the nanomedicine into the cytosol. This could be developed by carefully tuning lipid design to change their pKa in order to promote this effect.
This is another example which beautifully illustrates the importance of understanding how nanomedicines and targeted drugs are processed by cells so that their design can be carefully tailored in order to achieve the desired effects.
In order to characterize the intracellular trafficking of nanomedicines and targeted drugs, methods to be able to follow them inside cells over time and determine their intracellular location as well as the time needed to reach certain location are needed. For instance,
thwe
group have used live cell fluorescence microscopy imaging to measure nanoparticle location inside cells over time and in particular their colocalization with the lysosomes
[49][167]. Using nanoparticles of different sizes and theoretical modeling, the intracellular trafficking kinetics could be determined, thus how nanoparticle size affect the departure time from the cell membrane and arrival time to the lysosomes. With similar methods,
itwe can then
be determine
d how nanoparticle properties affect these details of intracellular trafficking, which also affect nanomedicine efficacy.
TheOur results also showed that within the same cells some nanoparticles arrive to lysosomes within short times, while others take much longer or get stuck somewhere else and never seem to arrive there. Additionally,
it iswe also found that not only uptake efficiency, but also intracellular trafficking kinetics strongly vary within individual cells in a cell population
[49][167]. Clearly, further studies are needed to understand similar observations and learn how to design nanomedicines and targeted drugs with controlled and uniform behavior at cell level. Within this
bac
kgroundontext, in order to try to understand the sources of such heterogeneity in nanoparticle uptake by cells,
the group rwe recently used repeated cell sorting to isolate cells with low and high nanoparticle uptake within a cell population and used transcriptomics to identify differences in gene expression in such sub-populations
[50][168].
Next to such imaging-based approaches, taking advantage of latest developments in high sensitivity flow cytometry,
thwe
group has have also developed a method based on organelle flow cytometry to gain temporal as well as spatial information on nanoparticle intracellular distribution and trafficking
[51][169]. The method allows to gain information on nanoparticle intracellular location as usually obtaining by imaging-based methods but with the high throughput and robust quantification enabled by flow cytometry. Thus, cells are exposed to nanoparticles, then all organelles are extracted and characterized by flow cytometry. In this way, intracellular trafficking kinetics can be easily obtained from thousands of organelles extracted from thousands of cells, without the complex analysis required to extract similar results from imaging-based methods. The two different methods present different advantages and limits, thus they can be used to complement each other in order to gain a better understanding on the details of intracellular trafficking of these object by cells. A better understanding of how cells process nanomedicines and targeted drugs is needed in order to optimize their design and efficacy.
4. Conclusdionng Remarks
Many new therapeutic entities with a beneficial pharmacological activity profile have an unfavorable distribution in the body, leading to toxicity or poor therapeutic effects. In fact, more than 90% of all new active substances that enter clinical phase I fail in subsequent clinical trials
[52][170] and 79% of these failures are due to safety or efficacy issues
[52][170]. The development of generic versatile drug delivery systems may therefore have profound effects in the pharmaceutical world and medicine. It may prevent the rejection of many potent drugs, and thus prevent loss of time and money. In parallel, it may open new opportunities for the treatment of cancer, chronic regenerative diseases, infections and other serious diseases with an unmet medical need.
An important group of drugs that may benefit from advanced delivery systems are biologics. Approximately 80% of all new biologics approved by the FDA (Food and Drug Administration) in 2020 are monoclonal antibodies
[53][171], and they represent 20% of all new FDA approvals. These can be considered an advanced drug delivery system in itself. However, many other biological compounds such as antisense oligonucleotides, RNA-and DNA-based therapeutics, peptides and proteins, will greatly benefit from a delivery system, not because of toxicity but in order to have a beneficial effect at all. Most of these more complex drugs have poor bioavailability and can easily be degraded. Additionally, they are too large to simply diffuse into cells, and are often poorly internalized, thus require a drug carrier for efficient internalization. More and more of these types of compounds, including peptides and oligonucleotides referred to as TIDES
[54][172], emerge in the list of FDA-approved molecules. They already represented 10% of all FDA approved drugs between 2016 and 2020
[54][172], underlining the need for further development of nanomedicines and drug targeting strategies. The recently developed RNA and DNA vaccines against COVID-19 infection have illustrated the potential of such novel types of drugs enabled by nanomedicine
[55][56][173,174].
Finally, nanomedicines as well as a drug targeting open many possibilities for a theranostic approach by delivering a diagnostic ligand and a therapeutic compound to the same cell or target site
[57][175]. Theranostics allow stratifications of patient groups before treatment and make early detection of disease activity and assessment of therapeutic efficacy of experimental drugs possible, which are all key hurdles in clinical trials, in particular for chronic fibrotic diseases
[58][176]. This type of personalized medicine may therefore represent an important innovation in medicine in the future.