Triads in skeletal muscle and dyads and peripheral couplings in cardiac muscle are the sites at which membrane depolarization is translated into Ca
2+ release from the SR and, eventually, muscle contraction. This process, called excitation–contraction (EC) coupling, requires the cross-talk between two main players: the ryanodine receptor (RyR), a highly conductive Ca
2+ channel embedded in the SR membrane and responsible for the rapid release of Ca
2+ from the SR, and the L-type Ca
2+ channel in the plasma membrane (T-tubule), which senses membrane depolarization and activates the RyR. The way RyR is activated differs in the cardiac and skeletal muscle systems. In the former, the opening of the cardiac muscle L-Type channel Ca
V1.2 generates a rapid Ca
2+ influx through the channel from the extracellular environment, causing a rapid increase in Ca
2+ concentration in the narrow space separating the T-tubule and the SR in the dyad, and inducing the opening of the cardiac RyR isoform, RyR2. This mechanism is defined as calcium-induced calcium release (CICR)
[12]. In skeletal muscle, the release of Ca
2+ from the SR is directly triggered by the activation of the skeletal muscle L-type channel, Ca
V1.1. The voltage-induced conformational change in Ca
V1.1 is mechanically transmitted to RyR1
[13], causing its opening. This mechanism, which is independent of Ca
2+ influx through Ca
V1.1, is known as voltage-induced calcium release (VICR).
In addition to the voltage sensor in the membrane (Ca
V) and the Ca
2+-releasing channel in the SR (RyR), the voltage-gated Ca
2+ channels β subunits are also essential for EC coupling
[14][15]. Ca
V1.1 and Ca
V1.2 channels bind to the skeletal β1a and cardiac β2a, respectively, through their alpha interacting domain (AID) located in the intracellular loop connecting transmembrane domains I and II
[16]. Beta subunits are crucial for facilitating the trafficking of the channel into the plasma membrane and for modulating the channel activity
[14][17]. In skeletal muscle, the adapter protein Stac3 is also required for voltage-induced calcium release
[18][19]. The exact role of Stac3 in skeletal muscle EC coupling is yet to be elucidated. Stac3 facilitates, but is not essential for, the membrane trafficking of Ca
V1.1
[20][21]; nonetheless, knocking out Stac3 completely abolishes the voltage-induced Ca
2+ release
[18][21]. The observation that Stac3 binds to the II-III intracellular loop of Ca
V1.1
[22], which is critical for the cross-talk between Ca
V1.1 and RyR1
[23], suggests that Stac3 might allow or facilitate the mechanical coupling between these two channels.
It appears evident that the association of all the EC coupling essential and accessory proteins and the efficient cross-talk between Ca
V1 in the plasma membrane and RyR in the SR require the accurate formation and organization of ER (SR)-PM junctions, making the proteins responsible for the organization of such junctions also essential for EC coupling.
2. The Junctophilin Family
Junctophilins (JPH1, JPH2, JPH3 and JPH4) were discovered by Takeshima and collaborators in the early 2000s in muscles and neurons
[24][25]. All four isoforms of this family contain a C-terminal transmembrane domain that embeds in the ER membrane and eight N-terminal domains called membrane occupation and recognition nexuses (MORN), thought to be responsible for the association with the plasma membrane (
Figure 2).
Figure 2. Schematic representation of junctophilin’s domain structure and its arrangement in the ER-PM junction. (
A) Linear map showing the first (I through IV) and second (VII to VIII) set of MORN domains (in pink) separated by the joining domain (white). The α-helical domain (orange) follows MORN VIII and is separated from the transmembrane (TM, in cyan) by the long divergent domain (green). The numbers underneath the map indicate mutations associated with human cardiomyopathies identified in JPH2 and their relative positions. (
B) Schematic representation of junctophilin’s organization in the ER-PM junctions (adapted from Garbino et al.
[26]). The MORN motifs associated with the internal leaflet of the plasma membrane and the C-terminal transmembrane domain embedded in the endo/sarcoplasmic reticulum membrane allow junctophilins to bridge the two membrane systems together. Different domains are color-coded as indicated in (
A).
3. Recruitment of Junctional Proteins by Junctophilins1 and 2
3.1. Recruitment of Skeletal Muscle Junctional Proteins
JPH1 and JPH2, and specifically a region in the two junctophilins spanning approximatively from the second half of the joining domain to the first half of the putative α-helical domain, co-immunoprecipitate with Ca
V1.1
[27]. A 20-residue sequence in the C-terminal domain of Ca
V1.1 is directly involved in the interactions with junctophilins and in the recruitment of Ca
V1.1 to triads
[28]. Additionally, Ca
V1.1 is recruited to junctions formed by JPH2 when the proteins are expressed in non-muscle cell models together with the Ca
V1.1 auxiliary subunit β1a and Stac3
[29]. Overall, this indicates that JPH1 and JPH2 have an active role in recruiting the voltage-gated Ca
2+ channel to triads by binding directly to the channel; the disruption of this interaction interferes with the assembly of the triad
[27][28].
JPH1 co-immunoprecipitates with RyR1, a behavior that has not been observed for JPH2
[30][47]. Nonetheless, JPH1 KO mice can still perform EC coupling
[30][31][32], suggesting that the presence of RyR1 in junctions does not depend solely on JPH1. Therefore, the recruitment of RyR1 in JPH2-induced junctions might be due to a weak interaction that is not detected in biochemical assays or requires the presence of additional proteins, with Ca
V1.1 being a likely candidate.
3.2. Recruitment of Cardiac Muscle Junctional Proteins
The cardiac L-type Ca
2+ channel Ca
V1.2 co-immunoprecipitates with JPH2
[32][33][34], indicating that, as in skeletal muscle, JPH2 likely plays a role in recruiting the voltage-sensor channel in the dyads and peripheral couplings of cardiac muscle. Notably, the same C-terminal sequence identified as the Ca
V1.1 site of interaction with junctophilins, is conserved in Ca
V1.2
[28], suggesting that this sequence might also be involved in Ca
V1.2–JPH2 interactions.
Differently from what was found with JPH2 and RyR1, co-immunoprecipitation was observed between JPH2 and RyR2
[32][33][34], indicating a stronger interaction between the two proteins. This interaction is disrupted by the E169K substitution located towards the N-terminal end of the JPH2 joining domain
[3534] and weakened by the R420Q mutation in RyR2
[3635]. A stronger interaction with RyR2 might be required by JPH2 because it is the only junctophilin isoform expressed in cardiac muscle and possibly because cardiac muscle lacks the additional stabilization provided by the mechanical connection between RyR1 and Ca
V1.1 that exists in skeletal muscle
[13].
4. Functional Studies on Junctophilins 1 and 2
4.1. Junctophilin 1
JPH1 knock-out mice die within 24 h after birth due to suckling defects leading to undernourishment. The suckling defect is likely due to muscle weakness since the neuronal suckling reflexes are normal in knock-out mice [37][48]. Functional studies on isolated hindlimb muscle showed abnormal twitch tension and a greater dependency on extracellular calcium in KO mice muscles, suggesting that a significant fraction of RyR1s in the junctional SR are not directly coupled with the CaV1.1 channels in the T-tubules and therefore operate via calcium-induced calcium release. Nonetheless, knock-out (KO) mice are still relatively mobile and show skeletal muscle-type EC coupling to a certain degree, indicating that JPH2 can support voltage-induced Ca2+ release in the absence of JPH1. From a structural point of view, although no major disorganization of the fiber is noticed at the light microscopy level, evident alterations are noticeable at the ultrastructural level [3130][3836]. In particular, the skeletal muscle of wt and JPH1 KO mice show a similar development in the embryonic stages until shortly after birth. At this age, wt muscle experiences a significant increase in JPH1 expression, which is temporally correlated with the transition from immature SR-PM junctions, mainly organized in dyads at this stage, into fully formed triads. This transition is absent in JPH1 KO muscle [3130][3836], suggesting that JPH2 is important in forming the dyads, while JPH1 has a crucial role in the conversion from dyads to triads in the fully mature skeletal muscle. The knocking down of junctophilins using sh-RNA, leads to the impairment of store-operated Ca2+ entry (SOCE), altered intracellular calcium release and intracellular calcium stores [3937] and to a reduction in RyR1 and CaV1.1 co-clustering associated with a decrease in CaV1.1 membrane expression [27] both in myotubes and muscle fibers. In both these studies, a shRNA against both JPH1 and JPH2 was used; hence, it was impossible to distinguish each isoform’s relative contribution to the resulting phenotype.
4.2. Junctophilin 2
JPH2 knock-out mice die in utero due to cardiac failure. Ultrastructural analyses on embryonic myotubes of KO mice revealed a substantial reduction in the number and extension of peripheral couplings
[25]. To avoid the complication related to the early mortality of KO mice, van Oort et al. generated a conditional JPH2 knockdown mice model to assess the effect of JPH2-reduced expression in the mature heart
[4038]. Inducing JPH2 knockdown led to an increased frequency of heart failure events. At the cellular level, this was explained structurally by T-tubule remodeling and destabilization and disorganization of the dyads, and functionally by Ca
V1.2 and RyR2 uncoupling and the consequent reduction in the efficiency of calcium-induced calcium release. An increase in the frequency of calcium sparks was noticed in knocked down isolated cardiomyocytes, suggesting that JPH2 might also modulate RyR2 by reducing its activity.
A number of point mutations in JPH2 have been discovered in association with hypertrophic cardiomyopathy and atrial fibrillation. The localization of these mutations spans from the N-terminal MORN motifs to the divergent domain at the C-terminus, indicating that multiple regions of JPH2 are involved in supporting cardiac muscle structure and function.
Amino acid substitutions N101R and Y141H in the MORN IV and VI, respectively, and S165F in the joining domain, cause similar phenotypes such as JPH2 mislocalization, reduction in spontaneous Ca
2+ signaling and increased cell size in HL-1 and H9c2 cell lines
[4139]. Mutations Y141H and S165F were also tested in skeletal muscle myotubes
[4240], where they were found to induce myocytes hypertrophy, reduce EC coupling gain and increase intracellular Ca
2+ concentration. Additionally, Y141H but not S165F pathogenically increased store-operated calcium entry
[4240]. Mutation E169K, located in the joining domain, causes weaker binding between JPH2 and RyR2 and increased spontaneous Ca
2+ leakage from the SR in the form of a spontaneous Ca
2+ release and increased Ca
2+ sparks in isolated cardiomyocytes from a pseudo-knock-in mouse model
[3534]. The A405S mutation is located in the putative α-helical region of JPH2. The equivalent mutation introduced in mice (A399S) resulted in cardiomyocytes with an irregular T-tubule pattern but otherwise relatively normal Ca
2+ signaling with only a moderate increase in sarco–endoplasmic reticulum Ca
2+ ATPase (SERCA) activity.
5. Post-Transcriptional Regulation of JPH1 and JPH2
Conditions of elevated cytosolic Ca
2+ concentrations lead to fragmentation of junctophilins 1 and 2 in skeletal and cardiac muscle. Murphy and colleagues
[4341] determined that exposure to elevated (≥20 µM) intracellular [Ca
2+] for 60 min led to the almost complete loss of full-length JPH1 and JPH2 in skeletal muscle fibers. This loss is mirrored by a loss of contractile force in skinned skeletal muscle fibers after just one minute of exposure to 40 μM Ca
2+. The same authors also observed fragmentation of JPH1 after raising the intracellular [Ca
2+] by supraphysiological stimulation of the muscle fiber. Interestingly, the proteolysis of JPH1 temporally matched the autolytic activation of calpain-μ (calpain1). The link between calpain, specifically calpain1, and JPH1 cleavage was recently confirmed by data from Tammineni and colleagues in patients with malignant hyperthermia susceptibility (MHS) and muscle cell lines
[4442].
After the identification of several putative calpain binding sites also in JPH2
[3433][4543], the implication of calpain in the proteolytic regulation of JPH2 was verified using calpain inhibitors to rescue the loss of JPH2 in an inducible heart failure mouse model and in mice cardiomyocytes after ischemia/reperfusion
[4644]. Akin to what was shown by Tammineni and colleagues in skeletal muscle, Guo and collaborators also observed that digestion by calpain1 releases several fragments of JPH2
[3433]. Among these fragments, a ~ 75 kDa N-terminal peptide (JPH2-NTP), generated by calpain1 cleavage at residues R565/T566 in the JPH2 divergent region, migrates into the nucleus, where it binds to TATA box regions and interacts with the transcription machinery
[4543]. Altogether, these results indicate a fine modulation of junctophilin 1 and 2 as a way to regulate intracellular Ca
2+ homeostasis and possibly reduce EC coupling gain in conditions of excessive intracellular Ca
2+ concentration.
6. New Insights from Deep Learning Protein Structure Prediction
Remarkable advancements in protein folding prediction were recently achieved by the artificial intelligence software Alphafold2 [4745]. Alphafold2 is a giant leap forward in the reliability of protein folding prediction compared to similar existing software [4846], and it has already been used to predict the structure of nearly the entire human proteome. Based on Aplhafold2 prediction models, junctophilin 1 and 2 show a similar 3D structure, also shared by the neuronal isoforms JPH3 and JPH4 (UniProt protein ID: Q9HDC5, Q9BR39, Q8WXH2, Q96JJ6 for human JPH1, JPH2, JPH3 and JPH4, respectively). The structure of the most ordered domains of junctophilin, specifically the MORN repeats, the α-helical region and the transmembrane domain, are predicted with high confidence by Alphafold2. In contrast, the joining and divergent domains are likely disordered, at least in the isolated protein, and the structure cannot be predicted with reasonable confidence. According to the prediction model (Figure 3), the MORN domains are arranged in an extended “halfpipe” configuration, with the α-helical domain lying on the convex side of this half-pipe (Figure 3B,C) and establishing interactions with charged residues in the MORN domains (see the zoomed-in region in Figure 3C for an example).
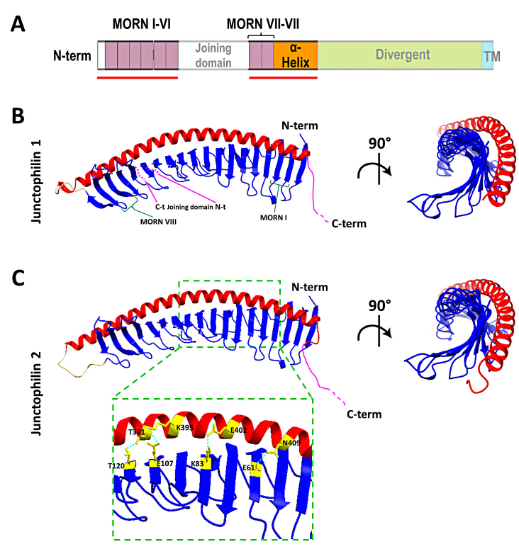
Figure 3. Structure of the MORNs and α-helical domains of human junctophilin1 and junctophilin2.(A) Schematic representation of junctophilin domain as shown in Figure 2; the solid red lines indicate the regions for which the structure is predicted with high fidelity by Alphafold2 and illustrated in (B,C). (B,C) predicted structures of the MORNs- -helical domains of junctophilin1 (B) and junctophilin2 (C). The α-helical domain (in red) lies on the convex side of the MORN domains half-pipe structure (in blue) in both junctophilin1 and junctophilin2. β-sheet hairpins forming MORN domains I and VIII (green parentheses) and the position of the N-terminal end (N-t) and C-terminal end (C-t) of the joining domain (in pink), which is absent in this representation, are indicated in (B). The inset in (C) shows some of the residues that form the hydrogen bonds that stabilize the association between the MORN domains and the α-helical domain of junctophilin2.
The structure obtained using Alphafold2 is substantially different from what was previously predicted using RaptorX software by Gross and colleagues [4947]. In the structure described by Gross et al., the α-helical domain extends beyond the MORN domains without interacting with them at all. However, Alphafold2 software is considered to be more accurate than most (if not all) of the currently existing structure-predicting software, especially for proteins for which no homologous structures exist [5048][5149], and the reciprocal arrangement of JPH2 MORN motifs and α-helical domain predicted by Alphafold2 agrees with data from Li and collaborators [5250] based on the crystal structure of the protein MORN4. MORN4 contains a series of MORN motifs arranged in a half-pipe configuration followed by a brief α-helical region. The helical region stabilizes the MORN domains by lying over part of the convex side of the half-pipe. The structure solved by Li and colleagues is in many ways very similar to the sequence predicted by Alphafold2 for JPHs.
Furthermore, in MORN 4, the concave side of the MORN half-pipe structure, containing most of the conserved residues that define the MORN domain, engages in the binding with the α-helical region of myosin3a. It is conceivable that the concave side of the junctophilin MORN motifs could also participate in protein–protein interactions with components of the EC coupling machinery. The particular arrangement of the α-helical domain with respect to the MORN motifs predicted by Alphafold2 and suggested by the observations of Li and colleagues challenges the classic view of the α-helical domain as the spacer that spans most of the junctional gap (see schematic representation in Figure 2) and points to the divergent domain as the region that most likely fulfills this role.