Animals adopt several strategies to regulate their body temperature by promoting heat loss or gain in hot and cold environments, respectively. This mechanism of heat loss or production is performed in thermal windows. A thermal window is a structure where many blood capillaries facilitate thermal exchange in this region. The presence of feathers, hair, or glabrous (hairless) skin and their structural characteristics greatly influence each species’ capacity to maintain thermal comfort. This factor needs to be considered when implementing new monitoring or measuring techniques such as infrared thermography since interpretations may vary due to the presence or absence of these structures. It is essential to recognize the effects of glabrous skin, hair, and feathers on thermoregulation to identify species-specific thermal windows that allow accurate evaluations of the thermal state of domestic animals.
1. Introduction
Thermoregulation is a process that responds to specific environmental conditions, such as heat or cold and can entail significant energy expenditures by individuals to maintain temperature homeostasis
[1,2,3][1][2][3]. These processes include a series of circulatory, physiological, behavioral, and metabolic adjustments to achieve the loss or retention of heat necessary to maintain correct cellular homeostasis and reduce the consequences that thermal stress implies
[4]. Thermal stress is an external condition that produces a strain in the biological functions of animals and is triggered when the environmental conditions exceed the critical range of temperature, requiring an increase in their basal metabolic rate to thermoregulate
[5].
This critical temperature range is known as the thermoneutrality zone, which is a “range of environmental temperature, specie-specific, at which thermoregulation is achieved solely by control of sensible heat without regulatory changes in metabolic heat production or evaporative heat loss”
[6].
Thermoneutrality is often associated with the thermal comfort zone; however, comfort is defined “in terms of perception and the state of mind that expresses satisfaction with the thermal conditions of the environment”
[7].
The responses triggered by animals outside the mentioned thermal zones involve various pathways in which the central nervous system (CNS) participates through the hypothalamus and the brainstem. On the other hand, the peripheral response is initiated by activating receptors specialized in recognizing thermal stimuli and the secretion of neurotransmitters secreted by the hypothalamus
[8]. Among these thermoreceptors, the Transient Receptor Potential (TRP) ion channels are the main receptors implicated in perceiving specific ranges of temperatures
[9]. Within the TRP family, two groups of ion channels are recognized for the perception of warm or hot temperatures (e.g., TRPV1-4, TRPM2, TRPM3, TRPM5) and cool or cold stimuli (e.g., TRPM8, TRPA1, and TRPC5)
[10]. The depolarization and action potentials produced by these receptors reach superior thermoregulatory centers in the hypothalamus, leading to physiological, behavioral, internal, and external mechanisms necessary to reach homeothermy
[11,12][11][12].
To avoid thermal stress, animals use three main mechanisms to thermoregulate
[11,13][11][13]. In the first instance, heat stress occurs in extreme environmental conditions, such as hot environments, resulting in an imbalance between internal demands and the environment, where the capacity to dissipate heat is altered
[14]. During heat stress, physiological mechanisms promote heat loss through cutaneous vasodilation and sensible heat loss by conduction, convection, and radiation due to the thermal gradient between animal and environment. Sweating and panting also increase evaporative heat loss when exposed to hot temperatures
[15,16,17][15][16][17] (
Figure 1). These mechanisms are essential, particularly for the Sphynx cat, the Xoloitzcuintli dog (Mexican hairless dog), and the Chinese Crested dog, which breeds characterized by a partial or entire absence of hair, classified as animals with glabrous skin
[18]. In the Sphynx cat, the presence of small and curved hair follicles with infundibulum and a hair bulb is recognized, but these follicles are in the continuous anagen phase, producing dysplastic hairs that cannot penetrate the epidermis and are not able to form a proper coat
[19]. On the other hand, in the Chinese crested dog, an autosomal dominant trait generates ectodermal dysplasia, deformities in the hair follicles, and condensation of mesenchymal cells, restricting the hair growth to the head, extremities, and tail
[19,20][19][20].
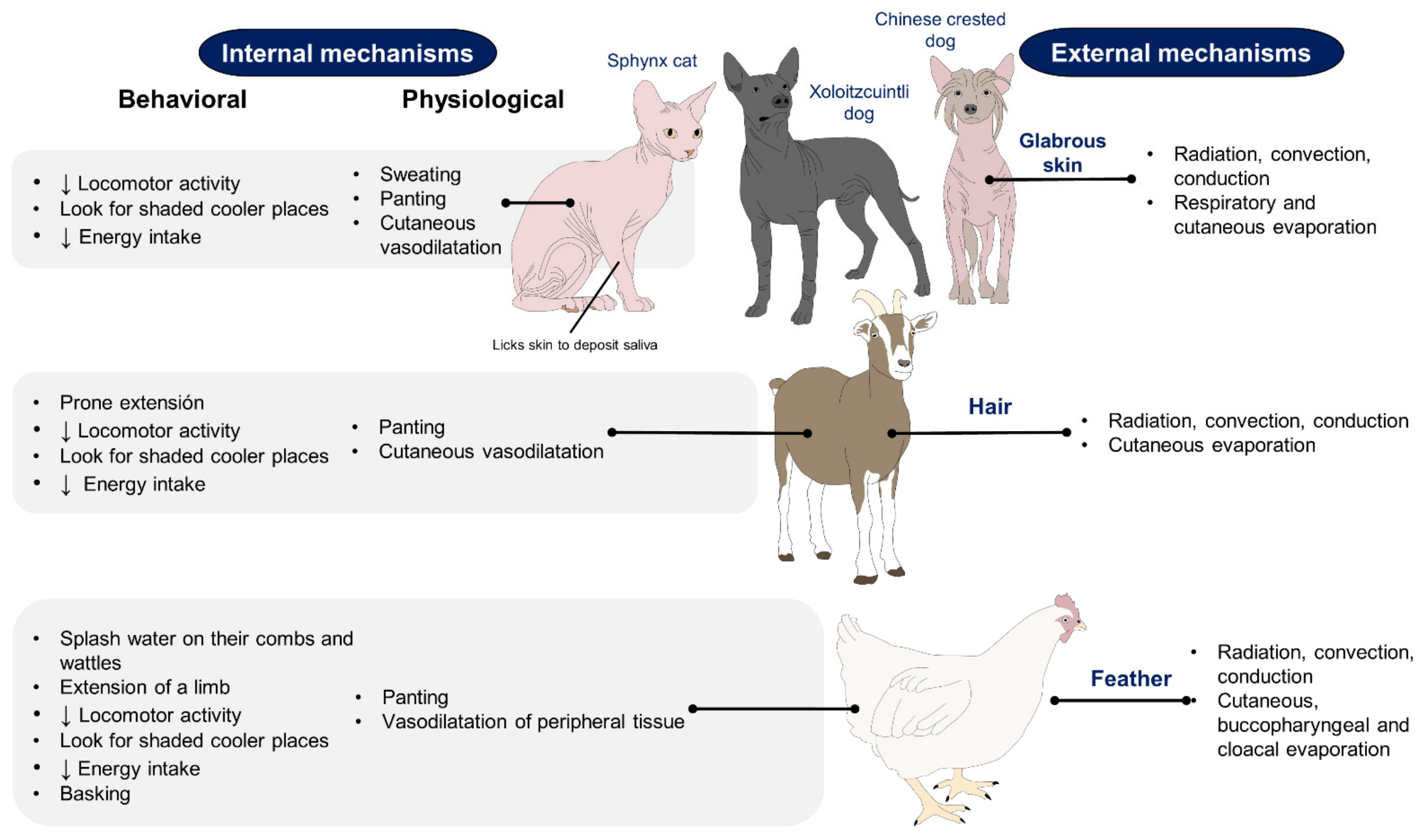
Figure 1. Compensatory mechanisms that animals with glabrous skin, hair, or feathers develop for hyperthermia avoidance. The morphological differences between species influence their behaviors and physiological responses to dissipate heat. Sphynx cats, Xoloitzcuintli, and Chinese crested dogs (seen in figure) sweat in the same way as other domestic animals. However, due to the bare skin, insensible perspiration is higher than other animals with hair or full coat and are predisposed to acute peripheral vasomotor responses to thermoregulate
[15,16,17][15][16][17].
Although these areas of glabrous skin have thicker epidermis and a more compact stratum corneum
[18], the absence of hair can alter the capacity of an animal to thermoregulate. Since hair is a physical barrier that intervenes in heat loss or heat retention, hairless animals are more sensitive to extreme temperatures and are predisposed to consequences such as sunburn when exposed to direct sun radiation
[21] and acute vasomotor responses
[22]. Additionally, in these species, particularly in the Xoloitzcuintli and the Crested dog, structural and functional defects are also present in the sweat glands
[20], motivating them to resort to specific behaviors such as saliva spreading onto the body surface to dissipate heat through evaporation
[23]. In contrast, responses to avoid hypothermia are activated when animals are under cold stress, where the temperatures are below the comfort zone, and the organism requires to increase its energy intake or metabolic production to maintain the body temperature
[24]. The main mechanisms to preserve or produce heat include increased activity, postural changes, shivering, and in some cases, non-shivering thermogenesis, peripheral vasoconstriction, piloerection, among others (
Figure 2)
[13,25,26,27,28][13][25][26][27][28].
Figure 2. Compensatory mechanisms that animals with glabrous skin, hair, or feathers develop for hypothermia avoidance. The morphological differences between species influence their behaviors and physiological responses to minimize heat loss
[26,27,28][26][27][28].
Some studies have suggested that infrared thermography (IRT) can aid in identifying responses to heat or cold by evaluating surface temperatures
[29,30,31][29][30][31]. This technique detects changes in the blood flow of the microvasculature in response to pathophysiological or environmental events such as heat or cold stress. As explained earlier, both situations trigger vasomotor responses of vasodilation or vasoconstriction, with the subsequent increase or decrease in the amount of heat dissipated and detected by thermography
[32,33][32][33]. However, the research conducted to date presents contrasting results because the regions useful for assessing temperature differ among animal species
[28,29,30][28][29][30]. Only limited information is available on thermal differences related to different types of body coverings—feathers, hair, glabrous skin—and extreme temperatures; for example, the relation between the consumption of energy resources and the type of brown adipose tissue (BAT) when animals are exposed to cold
[34], or circulatory changes in response to environmental heat
[35].
2. Effect of Hair, Feathers, and Glabrous Skin Based on Recent Scientific Findings on the Use of IRT
2.1. Hair
The presence and influence of hair on heat dissipation has emerged as the principal focus of many studies. A recent attempt to determine the effect of fur type on surface temperature evaluated 50 dogs with different coat types (curly, short, long, and double) by examining the entire left and right lateral regions. The animals with short fur presented a higher temperature (31.3 °C) than the dogs with long fur (28.3 °C) and the double layer (28.2 °C), while those with curly hair had an intermediate temperature (29.8 °C). This suggests that animals with a layer of short hair tend to present a higher surface temperature increase than those with a layer of long hair
[115][36]. These differences are presented in
Figure 63.
Figure 63. Effect of exercise (running for 15 min) on changes in dermal microcirculation in short- and long-haired dogs. (
A) rostral region of a short-haired dog at rest. The maximum nasal temperature (El1) is 26.3 °C, and the maximum right ear temperature (Li1, Li2, Li3) is 27 °C. (
B) rostral region of a short-haired dog after running. Despite the similar color pattern to thermogram (A), an increase in the maximum nasal (El1) and auricular temperatures (Li1, Li2, Li3) was recorded (3.8 °C and up to 10.8 °C, respectively). (
A’) rostral region of a long-haired dog at rest. The maximum muzzle temperature (El1) is 25.8 °C, 0.5 °C lower than in the short-haired dog. The maximum temperature of the right auricular (Li1, Li2, Li3) region is 21.6 °C. (
B’) rostral region of a long-haired dog after running. Compared to thermogram (
A’), an increase of 8.2 °C was registered in the maximum temperature of the nose (El1), seen in the color change in that region. In contrast, the temperature of the pinna (Li1, Li2, Li3) was 1.6 °C lower than in the short-haired dog (maximum value 36.2 °C). These findings suggest that the cutaneous vasodilatation response in the nasal region is greater in long-haired than short-haired dogs, while the latter present more evident vasodilation responses in the pinna. The higher surface temperature observed in dogs with short fur is mainly related to the closeness of the epidermis to the fur surface, which makes it possible to evidence more increased energy flow by longwave radiation. In the case of the dogs being under direct solar radiation, the situation could be inverse, depending on the thickness and color of the fur. Being darker will determine more significant absorbance and minor transmittance
[115][36].
2.2. Feathers
Studies of animals with feathers using invasive techniques have correlated circulating cortisol levels with the ocular region’s temperatures under caloric stress conditions (
Figure 74)
[31]. It should be noted that the ocular region is widely used in domestic animals to determine the increase in body temperature in response to the activation of the sympathetic nervous system under stressful conditions such as stress hyperthermia
[120][37]. This effect is observed as an increase in the superficial temperature of the orbital region
[121,122][38][39].
Figure 74. Potential thermal windows in birds. (A) Facial region: marked by a circle (El1) and limited to the orbital region, including the upper and lower eyelids. The cere and nares of birds (El2) are suggested as a potential window that participates in heat dissipation due to vascularization through the maxillary artery. Sp1 shows the default focal point of the software. (B) Plantar region: the tibial (Li1, Li2) and plantar region (El1, El2) receive vascular supply from the femoral and dorsal metatarsal arteries that contribute to thermal exchange. Sp1 shows the default focal point of the software. (C) Radial region: due to the presence of the radial artery that irrigates the axillary region (Sp1), this window incorporates the inner face of the wing (El1) into evaluations.
However, increases in the IRT of the orbital region have not only been associated with stressful stimuli. In studies in laying hens, it was observed that the animals exposed to environmental enrichment, to improve their well-being, the temperature of the ocular surface and the levels of corticosterone were higher, in comparison with animals that did not receive any enrichment
[123][40]. Regarding this, Jerem et al.
[124][41] mention that IRT, together with other techniques such as hormonal determinations, is an alternative to identify and correct potentially stressful situations for this species.
On the other hand, Powers et al.
[125][42] used IRT to assess passive heat dissipation in facial regions of various species of hummingbird (
Cynanthus latirostris,
Archilochus alexandri,
Eugenes fulgens) during flight, as well as in smaller species (
Lampornis clemenciae,
Selasphorus calliope). They observed that the latter had higher temperatures and lacked the mechanism of passive heat dissipation even when inactive. They also mention that this characteristic could justify using thermal shelters to benefit wild and domestic birds during rest to prevent heat loss
[126][43].
Loyau et al.
[127][44], in contrast, sustained that resistance to heat stress in domestic species can be improved through the genetic selection of laying hens. They evaluated heat dissipation through the wings based on 9355 IRT images which showed that the temperature of the shank presented higher estimates of heritability (0.22) than the wing (0.09) or comb (0.19). That correlation was attributed to the genetic selection of individuals. Additional essential data from that study revealed that the wing is a thermal window reflecting environmental temperature, so evaluations of featherless areas are necessary to determine the usefulness of IRT in this species and identify adequate thermal windows for its use.
2.3. Glabrous Skin
In the case of species with glabrous skin, interest centers on heat dissipation that occurs during exposure to cold. The principal concern with domestic pigs, for example, is the farrowing period when the presence of moisture can negatively impact neonates during the first hours of life
[128,129][45][46]. Wet surfaces have higher thermal conductivity coefficients, which leads to a substantial increase in the rate of heat loss of neonates, which have a high specific surface area and high conductance. Moreover, evaporation of moisture from the dermal surface and the resulting increase in heat loss can trigger hypothermia, which is detectable by IRT
[130][47]. This indicates a potential role for IRT in clinical and preventive veterinary medicine since it could be utilized in production units to reduce the mortality risks in this species.
For example, Schmitt et al.
[131][48] used the IRT to evaluate the neonatal survival of genetically selected piglets (animals with low residual feed intake and high residual feed intake). In 62 piglets, thermography associated with standard methods such as rectal temperature was shown to be a reliable predictor of postnatal thermostability in the first hour after birth, a period with high mortality rates due to hypothermia. Likewise, IRT identified that the animals with low residual feed intake had better adaptability to heat stress and that only the back and the ear base temperature correlated with rectal measurements.
3. Conclusions
Temperature, a vital parameter of all animal species and breeds, is influenced by diverse external and internal factors that modify organisms’ physiological and behavioral responses as they seek to adapt to different environments. In some species, structures of the integumentary system, such as skin, hair, and feathers, strongly affect the thermoregulation capacity of individuals and, as a result, the amount of heat they conserve or dissipate through their bodies. IRT, a tool that quantifies the infrared radiation emitted by a body, depends directly on these anatomical-morphological differences to objectively measure body surface temperatures in diverse species.
Because birds’ feathers function as insulators, measuring irradiated temperature in feathered zones is impossible. However, birds also have featherless areas such as the feet, beak, orbital region, and the head or breast of species such as vultures that can be considered potential thermal windows for evaluation. In contrast, hair, a characteristic of most mammals, presents diverse challenges due to variations in density, length, color, and form. This means that animals of the same breed but with different kinds of hair will have thermoregulatory mechanisms that are more or less efficacious than others. This fact directly affects IRT measurements.
In contrast, in hairless animals, the entire body may be considered a thermal window because microvascular changes in these species can be detected easily by thermography. However, this condition also makes them more susceptible to extreme climates since they do not have a layer to protect them from those conditions. Furthermore, in sweat-intensive glabrous animals, when subjected to active convective processes, they may show sufficiently high vaporization rates that can reduce skin surface temperature. For this reason, IRT has been proposed as a valuable technique for identifying hypothermia before hemodynamic imbalances can occur, potentially with severe repercussions for animal health.
Concerning the scope and perspectives for the use of IRT, based on the corpus of evidence consulted, we conclude that it helps evaluate the surface thermal responses that occur in response to changes in environmental temperature and in cases of pathology, intense exercise, and other states where it can function for early diagnoses in domestic animals. Finally, a note of caution: it is essential to consider each species’ specific morphological characteristics and physiological mechanisms to be evaluated.