Residential and commercial buildings are responsible for over 30% of global final energy consumption and accounts for ~40% of annual direct and indirect greenhouse gas emissions. Energy efficient and sustainable technologies are necessary to not only lower the energy footprint but also lower the environmental burden. Many proven and emerging technologies are being pursued to meet the ever-increasing energy demand. Catalytic science has a significant new role to play in helping address sustainable energy challenges, particularly in buildings, compared to transportation and industrial sectors. Thermally driven heat pumps, dehumidification, cogeneration, thermal energy storage, carbon capture and utilization, emissions suppression, waste-to-energy conversion, and corrosion prevention technologies can tap into the advantages of catalytic science in realizing the full potential of such approaches, quickly, efficiently, and reliably. Catalysts can help increase energy conversion efficiency in building related technologies but must utilize low cost, easily available and easy-to-manufacture materials for large scale deployment. This entry presents a comprehensive overview of the impact of each building technology area on energy demand and environmental burden, state-of-the-art of catalytic solutions, research, and development opportunities for catalysis in building technologies, while identifying requirements, opportunities, and challenges.
1. Introduction
Energy plays a vital role in modern society; however, it is also responsible for greenhouse gas emissions. Global energy consumption is on the rise driven by economic and population growth. As shown in Figure 1, although renewables are expected to become the primary resource, fossil fuels continue to increase, given the global demand increase [1].
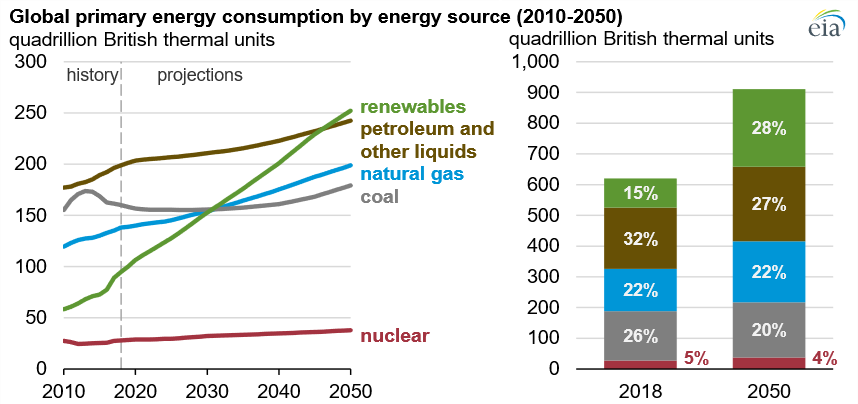
Figure 1. Global primary energy consumption by energy source, quadrillion Btu
[2].
By year 2050, energy information administration (EIA) projects a 50% increase in global energy demand driven by a 65% increase in building energy consumption, a 79% increase in electrical power generation, and a 40% increase in natural gas consumption
[2]. A continuous upsurge in energy demand due to increased affordability, economic growth, and new energy consumers poses a significant challenge to the energy infrastructure and the environment. Three major energy-consuming sectors in any economy are buildings, transportation, and industry. Amongst these energy consumers, residential and commercial buildings account for more than 30% of primary energy consumption. Combined direct and indirect carbon footprint of the buildings sector approached almost 40% of total carbon dioxide emissions
[3]. According to energy information administration statistics
[4], residential and commercial buildings in the USA consumed 41 exajoules (1.3 terawatt-year) of energy in the year 2019, accounting for almost 39% of total primary energy. As shown in
Figure 2, the electricity supply to the end consumer in the USA comes at a premium value since ~65% of the primary energy is lost in the process of production and distribution.
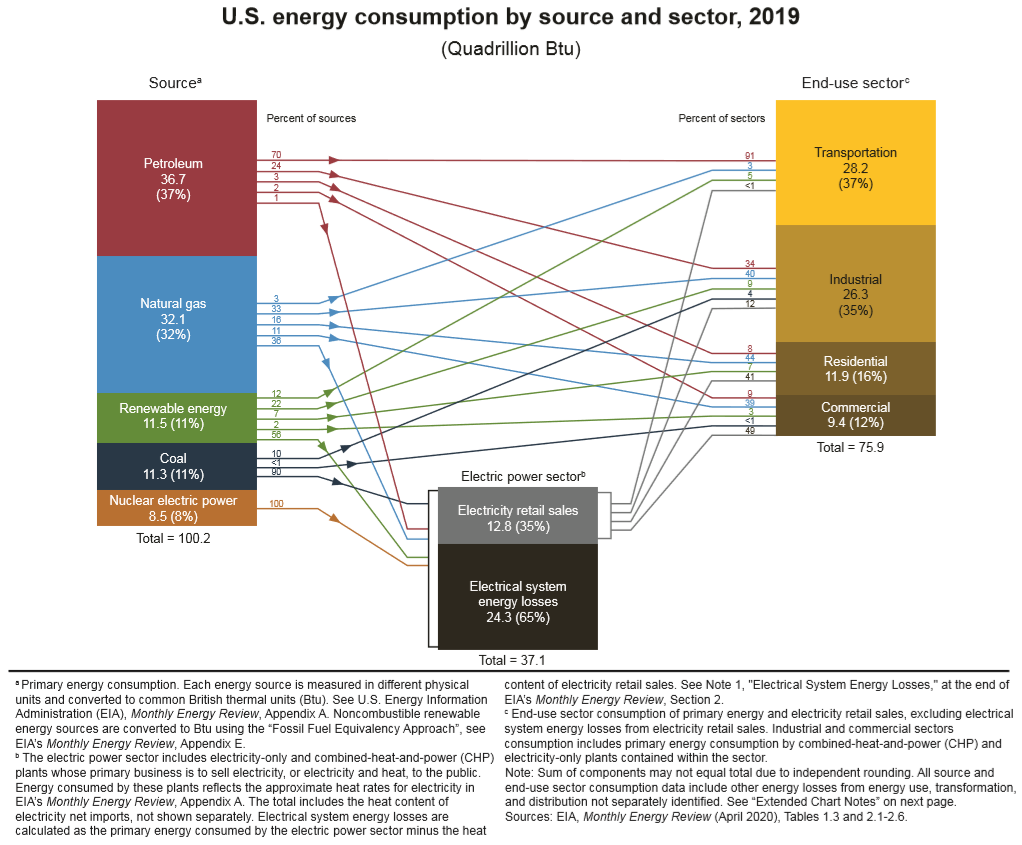
Figure 2. U.S. Energy consumption by source and sector
[4].
a Primary energy consumption. Each energy source is measured in different physical units and converted to common British thermal units (Btu).
b The electric power sector includes electricity-only and combined-heat-and-power plants.
c End-use sector consumption of primary energy and electricity retail sales, excluding electrical system energy losses from electricity retail sales.
Given this high premium, it is essential that the electric energy supplied to buildings is utilized to its full potential at the highest possible conversion and utilization efficiency. From an energy efficiency perspective, heating and cooling equipment in a residential or commercial building consumes up to 50% of the total energy supply
[5,6][5][6] in the USA. Heating systems, for instance, utilize ~60% fossil fuels as the primary energy resource
[7] in the USA, as shown in
Figure 3. As a result, building energy usage accounts for ~35% of total annual carbon dioxide emissions in the USA
[8], as shown in
Figure 4.
Figure 3. U.S. household heating systems’ energy source [7].
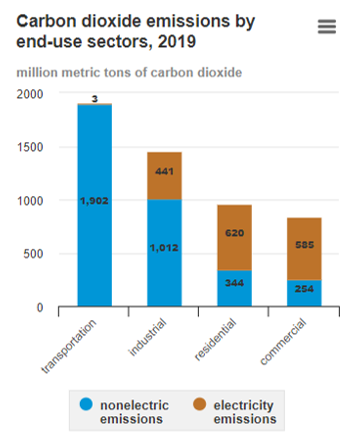
Figure 4. U.S. carbon dioxide emissions from energy consumption by sector
[8].
Given these energy and environmental impacts and projected statistics, technology improvements to address high energy demand, carbon footprint, and emissions associated with building energy consumption is of enormous value for a sustainable energy future. Additionally, buildings offer great potential to improve quality of life, health, and workforce productivity (defined as an assessment of the efficacy of worker(s)). For instance, a recent study by national institute of health
[9] focusing on the relationship between carbon dioxide, volatile organic compounds (VOC) concentrations, and associated the impact of air quality on cognitive function scores in office environments. The authors looked at nine different cognitive function domains as a function of carbon dioxide and VOC concentrations and found that clean and green buildings improve the scores by 50% to 200% depending on the activity domain.
Similarly, indoor air quality improvements are highly essential for the improved health of occupants in buildings. Various studies have established the significance of pollutant concentrations in homes and their impact on health and productivity of residents
[10]. A review of interactions between energy performance of the buildings, outdoor air pollution and the indoor air quality was recently reported
[11,12][11][12]. These studies investigated the impact of building energy efficiency improvements on pollutant concentrations and their health risk.
Increase in energy demand coupled with intermittent renewable energy resources in the grid infrastructure also emphasizes the importance of energy management and flexibility. For instance, efficient use of energy resources focusing on grid resiliency and environmental security supported by cogeneration and trigeneration technologies is a highly impactful approach. Numerous studies have already established the significance of such systems
[13,14][13][14]. These behind-the-meter assets have a significant potential in enabling sustainable energy technologies and can be deployed across the grid. Considering these factors, many opportunities exist in improving the health, productivity, energy efficiency, carbon footprint, and energy management for a sustainable future. Building energy resources such as heating, cooling, dehumidification, and thermal storage equipment, along with carbon capture/conversion, cogeneration and biomass conversion technologies play a vital role in realizing energy sustainability, occupant health and productivity, and environmental responsibility. In addition, energy efficiency, affordability, and retrofittability are some of the key attributes necessary for successful transition towards new technologies.
All these technologies utilize a vast array of chemical transformations, including (i) oxidation—for instance, pollutants, fuel conversion to syngas, and thermal storage, (ii) reduction—carbon dioxide conversion, (iii) hydration—thermal storage, (iv) dehydration—dehumidification, (v) absorption/chemisorption—heating and cooling, carbon capture, and (vi) hydrolysis and methanogenesis—biomass conversion to biogas. Given these chemical reactions and their potential for enabling sustainable societies, catalysis has a new significant role to play in buildings. In this context, the objective of this entry is to provide a comprehensive overview of the state of the art of catalysts in building applications, their impact, and future research and development opportunities. The next section introduces individual technology areas followed by a discussion on future directions for catalysts in enabling clean and sustainable energy in buildings.
2. Catalysts in Building Technologies
As discussed above, energy and environmental challenges associated with buildings can be overcome through the application of catalytic science. Many energy consumers within building envelope can exploit the advantages offered by innovations in the field of catalysis. Application of various catalytic reaction schemes shown in
Figure 5 include electrochemical, photochemical, photoelectrochemical, and thermochemical can aid in lowering the environmental and energy burden of the buildings. The rest of this section provides an overview of this.
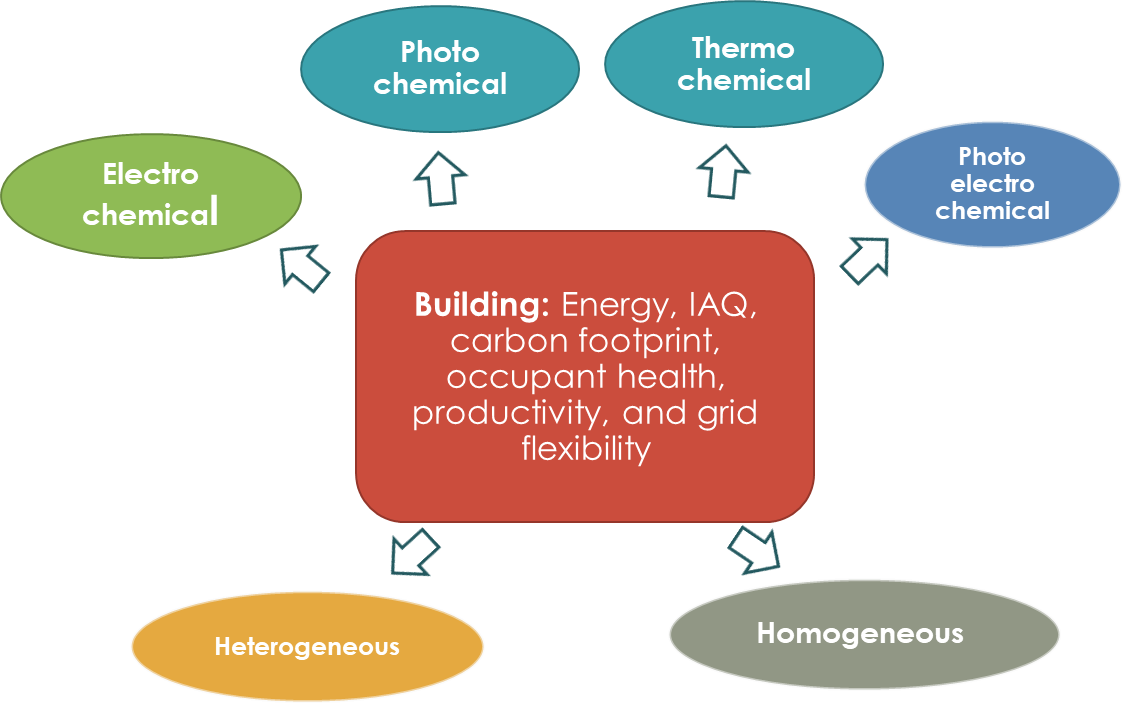
Figure 5. Opportunities for catalysis in buildings—sustainable energy, environment, and health.
2.1. Indoor Air Quality and Emissions
Common indoor air pollutants in buildings include volatile organic compounds (VOC), formaldehyde, benzene emanating from furniture, decoration, and personal care products
[15], along with combustion byproducts such as carbon monoxide, nitrogen dioxide, and sulfur dioxide from cooking and heating equipment. Energy efficiency improvements leading to tight sealing of the building envelope and lack of fresh makeup air in buildings can easily increase the concentration of these toxic compounds beyond what is generally observed outdoors
[16]. A review of standards and guidelines set by international bodies for the parameters of indoor air quality was recently published
[17]. Some of these threshold values are: 7 mg/m
3 over 24 h for carbon monoxide (CO), 0.1 mg/m
3 over 30 min for formaldehyde, 200 µg/m
3 over 1 h for nitrogen dioxide, and 0.5 mg/m
3 for total VOCs
[18].
Application of catalysts for mitigation of toxic indoor air pollutants has been extensively studied and reported
[19]. More recently, Gomez et al. applied a novel photocatalytic lime render for improving the indoor and outdoor air quality. This research team applied advanced lime-based binders with titanium dioxide (TiO
2) nano particles for the breakdown of formaldehyde via visible light and NOx pollutants via UV light
[20]. Similarly, photocatalytic binder-based mortars for renders and panels were applied to improve indoor air quality
[21]. Catalysts offer higher efficiencies at low operating temperatures for a wide variety of pollutants without producing any undesired byproducts. Photocatalytic oxidation using photosensitive semiconductors such as TiO
2 produces strong oxidizing agents to convert VOCs and formaldehyde into carbon dioxide and water
[22]. Supported metal oxide catalysts with enhanced catalytic activity was demonstrated via modified preparation procedure
[23,24][23][24]. Composite materials consisting of bioactive char and carbon nitride (C
3N
4) were shown to have a high formaldehyde conversion efficiency of 85%
[25]. Palladium (Pd) doped TiO
2 was shown to convert formaldehyde at ambient conditions
[26] and modified TiO
2/zeolite catalysts were highly effective in complete oxidation of benzene. Among different catalysts studied for the oxidation of VOCs and formaldehyde, TiO
2, cobalt (II, III) oxide (CO
3O
4), and alumina supported transition metal (Mn, nickel oxide (NiO
x), Fe) and precious metal (Pd, Ag) catalysts performed at high conversion rates exceeding 90%
[19]. Zeng et al. reported a multifunctional catalyst capable of oxidizing VOCs at high gas hourly space velocities of 594,000 h
−1 [27]. More recently, a review of catalytic oxidation of indoor air pollutants was reported
[28]. This study provided a comprehensive review of advances in oxidation catalysis for addressing harmful indoor air pollutants. Similarly, Boyjoo et al. provided a broad overview of photocatalysis, catalyst development as well as reactor design for implementing these catalysts for commercial air purification. Gas phase photodegradation of both VOCs and inorganic gases including ozone was reviewed in this study
[29]. Malayeri et al. conducted a thorough review of modeling approaches used in photocatalytic oxidation of VOCs. This study provided a broad overview of reaction mechanisms and kinetic models
[30]. Usage of photocatalysts in concrete as an environmentally friendly photocatalyst treatment methodology was reported by Nath et al.
[31]. Several studies looked at applying nonprecious metals as formaldehyde oxidation catalysts
[32,33,34][32][33][34].
Primary polluting and toxic constituents from gas fired equipment in buildings include carbon monoxide, nitrogen dioxide, unconverted hydrocarbons, and NOx emissions. Natural gas fired cooking and heating equipment in buildings can significantly impact indoor air quality if proper ventilation is not provided
[35]. Additionally, life-time performance degradation may also lead to undesired pollutants. Utilization of catalysts in building heating equipment to suppress these emissions is underexplored and is yet to enter the buildings market. NOx suppression via burner design has been heavily investigated although application of catalysts to tackle all criterion pollutants is sparse.
2.2. Dehumidification
Humidity control in buildings is an important research area with direct implications related to energy efficiency. Relative humidity (RH) in building environment plays a significant role in the health of occupants, building performance, and energy efficiency. The built environment can be severely impacted by high humidity. For instance, as shown in
Figure 6, high humidity levels beyond 65% can lead to condensation, mold/mildew growth, corrosion, equipment damage, building fabric deterioration, loss of insulation properties, discoloration, and potentially slip hazards
[36]. Dry environment, for instance RH below 20% can thrive atmospheres suitable for viruses and infection
[37]. Temperature of course plays a role in compounding the above issues further. Building energy performance, occupant comfort and health impact is well documented in several research studies. Vellei et al. studied the influence of relative humidity on thermal comfort
[38], where the authors analyzed and summarized designer friendly RH inclusive adaptive model to extend the range of indoor conditions for low energy naturally conditioned buildings globally. The impact of RH and temperature on structural properties of buildings was also reported
[39]. Similarly, a comprehensive review of indoor environment on mold contamination and hygrothermal effect was reported. The study analyzed the growth models in relation to temperature and humidity within the building envelope with a focus towards walls.
Figure 6. Relative humidity and temperature relationship in closed building environment—Comfortable vs. undesired ranges.
Air dehumidification, on the other hand, also has a significant potential in lowering the energy consumption during the space cooling processes. A reduction in latent cooling load via dehumidification helps decrease the energy consumption as well as it enhances the cooling comfort temperature range. In vapor compression systems (e.g., HVAC), latent cooling load could be as high as 50% of the total cooling load which is typically accomplished via cooling the process air below its dew point. Dehumidification can certainly lower or eliminate this load if it is accomplished via physisorption, chemisorption or electrolytic processes.
Electrolytic dehumidification using enhanced oxygen evolution reaction (OER) catalysts were employed in recent studies. For instance, Gao et al. utilized manganese (II, III) oxide (Mn
3O
4) supported on cobalt selenide (CoSe
2) catalyst for alkaline OER catalyst exhibiting superior performance
[40]. Stabilization of commercial iridium oxide (IrO
2) catalysts via usage of transparent oxides, such as tin doped indium oxide and fluorine doped tin oxide was employed
[41,42][41][42]. Antimony pentoxide (Sb
2O
5):Tin oxide (SnO
2) was also employed as a support material in improving the stability and conductivity of OER catalysts. Li et al. studied different structurally modified OER catalysts via nanodendrites as supports for IrO
2 catalyst where the Polymer electrolyte membrane-based dehumidification was enhanced by 45%
[43].
Photocatalysts for dehumidification of ambient air is another approach employed by few researchers. Carbon nitride catalysts were studied for dehumidification and hydrogen evolution reaction to develop metal free catalysts for photoelectrocatalysis and electrocatalysis
[44]. Similarly, tantalum nitride (Ta
3N
5) nanorod crystals grown on potassium tantalate (KTaO
3) particles were also studied for generating hydrogen via photocatalytic splitting of water
[45]. More recently, Yang et al.
[46] developed a novel hybrid system consisting of Zn/Co based superhygroscopic hydrogel incorporated with copper oxide (Cu
2O) and barium titanate (BaTiO
3) nanoparticles for dehumidifying air.
Improved performance of silica gel by employing promoters such as Al, Co, and Ti ions was also reported
[47]. This study showed improved adsorption capacity, stability, and longevity due to the formation of Si-O-M (M = promoter element) linkages in the doped silica gel. Similarly, several researchers also enhanced the performance of silica gels by adding promoters such as calcium chloride and other halide complexes
[48,49,50][48][49][50].
2.3. Thermal Energy Storage
Energy storage is critical to enable integration of intermittent renewable energy into grid infrastructure. A significant portion of peak demand consumption in buildings is associated with thermal energy (as high as 40%). Storage and utilization of thermal energy is of great value in peak shaving applications. Thermal energy storage via utilizing sensible and latent storage technologies is very well studied. However, thermochemical energy storage offers higher energy density due to high amount of enthalpy change associated with reversible chemical reactions
[51[51][52],
52], as shown in
Figure 7.

Figure 7. Comparison of different thermal energy storage technologies’ energy storage density and reaction temperature.
In reversible thermochemical reactions, heat is stored during endothermic reaction and released during the reverse exothermic reaction. A review of high temperature thermochemical reactions was recently published where the concepts and reaction mechanisms of several classes of reversible chemical reactions are discussed
[53]. Thermal storage with metal hydrides is an attractive option for moderate thermal energy storage. Several reaction and catalytic strategies to enhance thermal storage capacity and stability of metal hydrides was recently published
[54]. Magnesium hydride (MgH
2) coated with iron, vanadium, chromium catalysts was shown to improve the reaction kinetics and thermal conductivity of MgH
2 [55]. Additionally, Titanium based catalysts were shown to enhance the reversible hydrogen release and uptake in sodium-aluminum hydride complex
[56].
Dry reforming of methane using solar thermal power plants is an attractive option for thermochemical energy storage due to its high endothermicity (259 kJ/mol). Several studies provided a comprehensive review of catalysts for enabling this reaction
[57,58,59][57][58][59]. Alumina-supported bimetallic Pt-Ru catalyst was recently utilized in dry reforming of methane as a thermochemical energy storage medium where the authors showed a stable performance for continuous cycling in a closed loop application
[60].
Iron (III) oxide was utilized as a catalyst and heat transfer medium in enabling a sulfur trioxide (SO
3) to sulfur dioxide (SO
2) reversible thermochemical dissociation reaction for storing concentrated solar thermal power at 850 °C
[61]. This study showed the significance of sintering temperature of the catalytic particles in enhancing the stability and cyclability. Similarly, calcium oxide redox couple with carbon dioxide has been extensively researched for applications in thermal energy storage owed to its high endothermicity (178 kJ/mol). Enhancement of the material’s optical properties, catalytic properties, and cycling stability via promoter elements such as iron and manganese was shown in a recent study, achieving 2.5 MJ/kg of energy storage density
[62]. Ammonia based thermochemical thermal energy storage with a reaction enthalpy of 66.8 kJ/mol is another area of high interest when utilizing concentrated solar thermal power.
Although several reaction schemes are possible for thermal energy storage, moderate- to-low temperature reactions are highly desirable for applications in buildings. Salt hydrates, hydration, and hydrogenation of metal oxides with promoters such as rare-earth metals and ternary compounds with transition metals or alkaline earth metals have been shown to enable these reactions, while improving stability and cyclability. Ideally, reactions capable of utilizing low grade waste heat while supplemented with renewable solar thermal energy all potential candidates for applications in certain climatic regions.
2.4. Carbon Dioxide Capture and Conversion
As described in the introduction section, buildings account for 40% of total carbon dioxide emissions globally (including electricity generation and onsite fuel consumption). The capturing and storage or conversion of this carbon dioxide to useful products is an underexplored area in the context of buildings. Post-combustion carbon capture technologies are suitable for removing carbon dioxide from flue gas generated by fuel combustion in furnaces, boilers, and water heaters. Additionally, direct air capture technologies are suitable for removing the carbon dioxide from air stream recirculated within the building. For instance, a residential building consuming 12 kWh/day of thermal energy can produce up to 2.1–2.5 kg/day of carbon dioxide, depending on the thermal efficiency of the heating equipment. Various materials have been explored to capture carbon dioxide, including metal oxide frameworks (MOF)
[63,64][63][64], carbon fiber
[65[65][66],
66], ionic liquids
[67[67][68],
68], activated carbon
[69[69][70],
70], non-carbon solid sorbents
[71], aqueous sorbents
[72], and organic membranes
[73].
Mukherjee et al. reviewed the application of activated carbon for post-combustion removal of carbon dioxide from flue gases
[74]. The application of photocatalysts based on carbon nitrides (CN) and their enhancement with inorganic semiconductors, carbon materials, ruthenium catalysts, MOFs, and porous materials to promote gas adsorption was reviewed by Liu et al.
[75]. In the same study, phosphorus doped carbon nitride-based nanotubes were also investigated for the photocatalytic reduction in CO
2 to enhance electrical conductivity and photo-reactivity. This study concluded that phosphorus doping enhances charge separation, surface area, adsorption capability, and morphology to improve the overall carbon dioxide reduction activity.
Conversion of carbon dioxide into useful products via many chemical transformation routes including electrocatalysis, photothermal catalysis, and thermocatalysis has gained significant momentum in the last two decades, owing to the climate change urgency. Such mechanisms will not only suppress the direct carbon footprint, but also negate the indirect carbon dioxide emissions associated with producing chemicals at the production source (e.g., methanol—industrial chemical; potassium carbonate—glass, soap industry; etc.).
Amplification of photothermal heterogeneous catalysts for valorization of carbon dioxide has been recently reviewed
[76]. A comprehensive interpretation of the heat-light interaction and directions for CO
2 utilization via novel photothermal catalyst design was provided. CO
2 hydrogenation via black indium oxide as a photothermal catalyst was also reported
[77]. Enhancement of light absorption by making yellow indium oxide into black color was synthesized and reported, showing 100% selectivity towards the hydrogenation of CO
2 to carbon monoxide with a turnover frequency of 2.44 s
−1. Similarly, Jantarang et al. investigated the role of support in photothermal hydrogenation of carbon dioxide using ceria-titania composite supported nickel catalyst
[78].
Thermocatalytic conversion of CO
2 to useful products is a highly attractive consideration if the net CO
2 output is not increased as a result of the conversion process. Usage of renewable energy to enable these reactions would be the desirable pathway, as shown in
Figure 8. Some of the possible reaction schemes are listed below:

Figure 8. Catalytic reactor scheme to convert CO2 from buildings to produce useful products. 1—carbon dioxide source, 2—other reactants, 3—renewable energy source, 4—gaseous products, 5—liquid products.
Developments in the application of heterogeneous catalysis for CO
2 utilization as a feedstock to produce fine chemicals and renewable fuels was reviewed by De et al.
[79]. This is an excellent study providing a very broad overview of thermodynamic considerations in designing a suitable catalyst for CO
2 activation and reaction on several classes of heterogeneous catalytic compositions. Additionally, a literature review of all possible reaction schemes and catalyst options was conducted and reported. Reactions schemes in this study included the production of CO via hydrogenation and the production of alcohols and hydrocarbon chains. Some of the catalyst options for CO
2 hydrogenation reported include (i) noble metal catalysts and their combinations as bimetallic catalysts with Co, Ni, Au, K (ii) supported Cu, Fe-Ni, Ru-Fe, La-Fe, Fe-Ni-Zn catalysts, (iii) support materials including zeolites, molybdenum carbide (Mo
2C), ceria, alumina, ceria-alumina (CeAl), SiO
2, doped ceria, etc. GHSV varied from 30,000 to 300,000 mL.g
ca−1h
−1 depending on the catalysts employed, while the selectivity and conversion rates varied significantly. Ethanol synthesis catalysts included precious group metals (PGM) and non-PGM catalysts supported on iron(II, III) oxide (Fe
3O
4), TiO
2, Co
3O
4, MCM-41, etc. and the reported GHSV was in the range of 6000 to 30,000 mL.g
ca−1h
−1. A non-exhaustive list of catalysts for the production of multi-carbon hydrocarbon chains from CO
2 was also reported. These reactions were enabled by non-PGM, transition metal, rare earth metal catalysts including Fe, In, K, Zr, Zn, Na, Ga, etc. supported mostly on nano tubes, ZSM, and SAPO.
The electrochemical reduction in CO
2 using various electrocatalysts has been recently reviewed in a comprehensive manner. Possible ways of converting waste CO
2 into useful products and thus contributing to the control of global warming while also producing new materials was thoroughly reviewed. Conversion of CO
2 to diverse products such as formic acid, carbon monoxide, methane, and methanol using flow cells, high pressure strategies, molecular catalysis, high temperature solid oxide electrolysis, and non-aqueous electrolytes was reviewed. The authors emphasized the significance of understanding the mechanism including that of poisoning for maturing these technologies in realizing carbon neutral cycles
[80,81][80][81].
Similarly, evolution of the electrocatalytic reduction in CO
2 from 2004 to 2018 was reviewed by Lee et al. The authors identified the bottlenecks for large scale implementation of the technology and concluded that the gas phase electrolysis offers a beneficial approach
[82]. Application of two-dimensional materials as electrocatalysts in reducing CO
2 as opposed to 3D structures was reviewed by Zhu et al. where the authors provided theoretical insights into the advantages of 2D approach such as improved electrical conductivity, larger surface area and the ability to access abundant surface active sites, etc.
[82]. Another prominent approach in electrocatalytic conversion of CO
2 via ionic liquids was recently reviewed by Lim et al. A review of experimental and theoretical investigations in to room temperature ionic liquids (RTILs) was provided, owing to their high selectivity and efficiency benefits
[83].
2.5. Heating and Cooling
Thermal energy in buildings is a significant energy burden. Considering all the energy needs in a typical residential or commercial building, heating and cooling equipment consume up to 40% of the total energy supply. Utilization of low-quality thermal resources in upgrading the heat to higher temperatures suitable for buildings, via heat pump technology is a highly effective method.
Electrically driven heat pumps use a vapor compression cycle to upgrade the heat for occupant comfort in a building, by harvesting ambient thermal energy. Energy resources for such heat pumps include geothermal, ambient air, and surrounding water bodies which act as heat sources to enhance the thermodynamic cycle performance in providing both heating and cooling energy. Other heat pump approaches involve absorption or solid–gas adsorption cycles to lower primary energy consumption and greenhouse gas emissions by utilizing waste heat or solar thermal energy. Fossil fuel combustion-driven heat pumps (thermally driven heat pumps) can also achieve coefficient of performance (defined as useful thermal energy produced vs. primary energy consumed) above 1 (1.2–2.5 in heating mode and 0.8–1.6 in cooling mode) but are lower than electrically driven vapor compression cycles.
Waste heat as the primary energy resource can offset the overall carbon footprint associated with thermal production. The working principle involves evaporation and consecutive absorption or adsorption of a refrigerant/coolant on the medium, as shown in
Figure 9. Absorption cycles typically employ lithium halide/water and water/ammonia as the working fluid pair. Adsorption cycles utilize physisorption on a solid surface where the working fluid evaporates producing cold for cooling or heat is extracted from a low temperature source in heat pump application. Adsorption heat pumps utilize microporous materials such as zeolites
[84[84][85],
85], aluminophosphates
[86], porous polymers
[87], and metal oxide frameworks (MOF)
[88].

Figure 9. Depiction of a thermally driven heat pump cycle providing thermal comfort in a building.
Compared to the above sensible heat technologies, chemical heat pumps offer higher energy density due to high heat of reaction, as discussed in the thermochemical thermal energy storage topic 2.3 above. The chemical heat pump composes of an endothermic reactor absorbing low temperature heat and an exothermic reactor releasing high temperature heat by utilizing reversible thermochemical reactions. Such a system typically consists of a condenser, an evaporator, and a reactor or adsorber coupled with a generator. It is used for upgrading and storing low grade heat via reversible chemical reactions.
Wongsuwan reviewed chemical heat pumps and their applications in using low grade waste heat
[89]. Dehydrogenation of isopropanol over Raney nickel (RN) catalyst
[90] was extensively studied and reported for a rapidly quenched and standard RN catalysts. Advances in organic liquid-gas based chemical heat pumps assisted by catalysts was reviewed by Cai et al.
[91]. Some of the reactions and catalysts reviewed included dehydrogenation of isopropanol and hydrogenation of acetone (carbon-supported PGM, Raney nickel, metal oxide-supported Cu), dehydrogenation of cyclohexane (Pt/Al
2O
3), dehydration of tert-Butanol (ion exchange resins), depolymerization of paraldehyde (solid acid). This study also highlighted the advantages of chemical heat pumps compared to vapor compression cycles. Additionally, a detailed overview of problems and future directions concerning chemical heat pumps was provided.
2.6. Cogeneration
The simultaneous production of electrical and thermal energy (heating or cooling) to offset energy consumption in a building is of significant value in the context of primary energy consumption reduction, grid flexibility, carbon footprint reduction and operational cost savings
[13]. Thermal energy and electrical energy generation from the prime mover can be stored and utilized via hydronic systems (to provide heating) and dehumidification (to reduce the latent cooling load, cooling). The produced electrical power can directly support the load profile and/or stored for later consumption via batteries or exported to the grid. An approach to utilizing a cogeneration system in a building is displayed in
Figure 10.
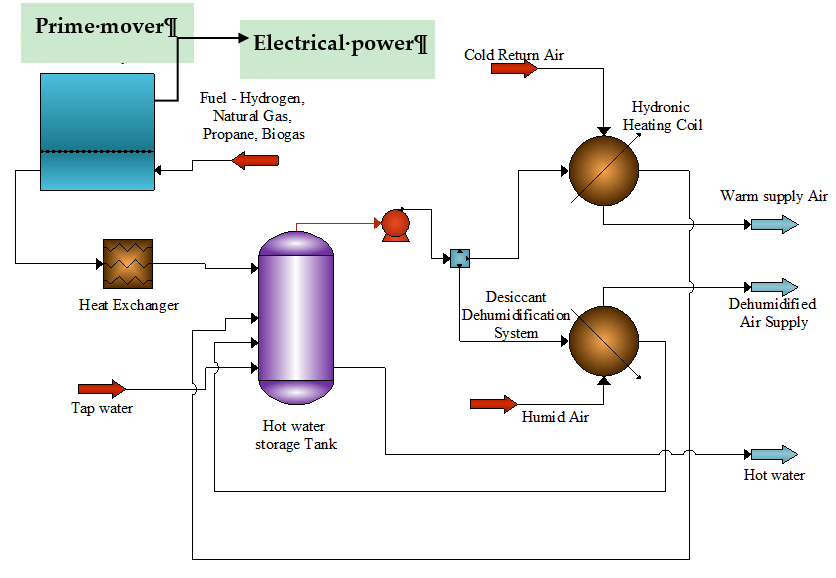
Figure 10. Cogeneration system using a prime mover to provide electrical and thermal energy in a building.
Fuel-driven prime movers suitable for building energy include polymer electrolyte fuel cells (PEM), solid oxide fuel cells (SOFC), reciprocating engines, thermoelectric, Stirling engines, etc. Some of these technologies may not be suitable for building applications due to the physical footprint and other integration challenges. These technologies also act as bridging solutions for enabling low carbon building energy while the electric power grid transitions towards higher efficiencies and composes mainly of clean renewable resources, such as solar and wind power.
The role of catalysts in prime mover technologies is well established. Three main classes of catalysts are utilized in these systems viz. fuel processing (including reforming, purification, and poison removal), emissions suppression, and electrode catalysts. Such catalysts are either employed in thermo-catalytic or electrochemical or electro-thermo-catalytic reactions.
Fuel processing catalysts enable the reforming of hydrocarbon fuels to generate syngas via partial oxidation, steam reforming, dry reforming, autothermal reforming for SOFC applications and downstream purification of hydrogen via water-gas shift reaction, and preferential oxidation for hydrogen generation in PEM fuel cell applications. This catalyst class also includes the removal of poisonous compounds from fuel (for instance, sulfur compounds from fuel). Emissions suppression catalysts help remove undesired toxic components such as carbon monoxide, nitrogen oxides, sulfur dioxide, etc. from the flue gas to enable clean fuel oxidation and release into the atmosphere. The primary function of an electrocatalyst is to oxidize the fuel (anode half-cell reaction) and reduce oxygen (cathode half-cell reaction).
A comprehensive review of reforming catalysts for hydrogen generation in fuel cell applications was provided where the authors reviewed reactions and catalyst systems for syngas generation
[92]. Several review articles focusing on reforming catalysts were published in the last five years and some of them are listed here, as follows: methanol steam reforming catalysts
[93[93][94],
94], non-precious metal-based ethanol steam reforming catalysts
[95], dry methane reforming catalysts
[58], bio-oil steam reforming catalysts
[96], dry reforming catalysts
[97], biomass pyrolysis oil steam reforming catalysts
[98], partial oxidation reforming catalysts
[99], and nickel-based methane steam reforming catalysts
[100].
Lehtoranta et al. recently reported the use of two different catalysts in natural gas emission reduction strategies from engines
[101]. Another study investigated the catalytic oxidation of methane fuel in the exhaust gas of a lean-burn natural gas engine
[102]. Similarly, Gremminger et al. investigated PGM catalysts for the oxidation of methane and formaldehyde in the exhaust gas, where the authors concluded that Pd-based catalysts were suitable for methane oxidation in lean conditions, while Pt catalysts were suitable for stoichiometric conditions. Additionally, an alumina-supported Pt catalyst was shown to provide excellent formaldehyde oxidation activity but high sensitivity to CO poisoning. Several research studies also investigated and reviewed the application of novel catalysts for the removal of NO
x and SO
2 from flue gas
[103,104][103][104].
The field of electrocatalysts is a heavily investigated area due to its implications in carbon dioxide conversion, automotive and stationary power generation, distributed power solutions, etc. Sui et al. recently provided a comprehensive review of Pt electrocatalysts for oxygen reduction reaction (ORR)
[105], while Ioroi et al. and Yang et al. reviewed a wide range of electrocatalysts for PEM fuel cell applications
[106,107][106][107]. Several studies also looked at recent developments in electrocatalyst design with and without noble metals
[108,109][108][109]. An interesting study by Ma et al. recently concluded that novel ORR electrocatalysts in PEM fuel cells via introduction of heteroatoms and defects into carbon materials (e.g., N-doped carbon nano tubes CNT) offer significant promise in enhancing the reaction kinetics
[110].
Development of porous SOFC electrode structures with enhanced oxygen reduction reaction at lower operating temperatures has taken a front seat in the last decade. The primary objective of these research efforts is to extend triple-phase boundaries while enhancing the ionic and electronic conductivities. Detailed reviews of several classes of cathode materials have been published: core–shell structures
[111], composite materials
[112], Ruddlesden-Popper perovskites
[113], perovskite oxides
[114].
Similarly, several researchers investigated anode electrode modification for direct internal reforming and enhancing carbon and sulfur tolerance by incorporating dopants and catalytic functional layers
[115,116,117,118][115][116][117][118]. A comprehensive review of recent advances in the development of anode materials for direct hydrocarbon fuel conversion was provided by Shabri et al.
[119]. This study focused on carbon deposition issues related to direct reforming on Ni and Ni alloys with several transition metals and made a recommendation for a hybrid mixed oxygen carrier perovskite and Ni/Ni alloy as a suitable anode material for enhanced carbon tolerance.
3. Discussion
In the field of indoor air quality and emissions, there is an absolute need for highly active, non-PGM catalytic materials which are tolerant to poisoning species. Incorporation of these materials into reactor structures offering negligible pressure drop with high capacity (lifetime enhancement) and ability to integrate in existing building stock is a critical requirement. Catalytic materials capable of addressing multiple reactions with high selectivity would be ideally suited for building air quality improvements. Such catalysts must be capable of suppressing CH4, CO, NOx, formaldehyde emissions from the flue gas generated from gas fired heating, and cooking equipment as well as from prime movers in cogeneration systems. Photocatalyst based solutions could play a vital role in successful implementation of such solutions. Buildings offer a variety of surfaces where such systems can be incorporated, for instance, selective surfaces within the building envelope. Hybrid materials with adsorbents and catalysts are necessary for improved conversion and selectivity.
Primary energy consumption reduction by lowering the latent cooling load via dehumidification of return air or fresh air supply in building cooling system also relies on some of the above-mentioned features necessary for successful application in residential equipment. Economic and population growth coupled with affordability are poised to drive the energy consumption associated with cooling needs in a building higher. The ability to incorporate dehumidification strategies in the air conditioning unit requires high capacity, selectivity, and lower regeneration energy. Another novel area with high potential for future deployment in buildings include dehumidification coupled with power production using advanced hybrid photocatalytic materials.
Thermal energy storage is another high impact area where the beneficial features of catalytic materials can be employed in enhancing the ability to use different heat sources, including low grade waste heat and renewable solar thermal energy, more effectively. Intermittent renewable energy sources, such as wind and solar integration at a grid scale, require energy storage technologies to offset the unpredictability by storing and using the energy at low cost. Successful deployment of high energy density thermal energy storage technologies enabled by heat of reaction from a reversible chemical reaction can help address grid flexibility and energy management. Catalytic promoters and additives to address the cyclability and stability of thermal energy storage materials can certainly help bring these solutions in to the real world.
Carbon dioxide emissions suppression from existing buildings’ gas fired heating/cooking equipment requires affordable, retrofittable, and easy to maintain solutions in capturing CO
2 and converting to useful products. Products from carbon conversion however requires a logistical chain to handle the derivatives appropriately. Ideally, a locally consumable product (e.g., fertilizer) would be beneficial to lock in the carbon. Co-adsorption of CO
2 and steam from the flue gas followed by in situ chemical transformation on a photocatalyst is a highly desirable route for carbon management in a building. Fu et al. recently published an article focusing on such an approach
[120]. Another useful approach could be the incorporation of catalytically enhanced CO
2 sorbents into the duct work of buildings as a long-term storage solution; however, the adsorption–desorption kinetics will have to be considered carefully. Metal support interaction phenomenon observed in heterogeneous catalysis may help address and tailor the reaction schemes such that CO
2 concentration does not pose an issue during transient cycles. Similarly, the indoor emissions and air quality improvements could be accomplished with the same duct with targeted materials placed strategically in each zone of the building (e.g., kitchen vs. rest of the building). Additionally, carbon dioxide capture should not impose further energy burden in a building. Hence, the usage of renewable energy and application of photocatalysts is desirable in enabling such chemical transformations without energy penalty. Song et al. recently proposed a hybrid approach for CO
2 capture
[121].
Non-precious metal-based, earth abundant, durable, easy-to-process-and-manufacture catalysts are needed for integration with cogeneration technologies. Fuel processing catalysts which can suppress carbon deposition along with being tolerant towards sulfur species are necessary. SOFC-based electrocatalysts capable of operating at lower temperatures in the range of 500 °C can enable affordable power generators with low capital expenditure costs.
In conclusion, implementation of catalytic technologies in buildings is needed to improve our health and workforce efficiency or productivity, lower the building energy consumption, and lower its carbon footprint. Catalysts have a new role to play in enabling sustainable energy future to meet the growing energy needs of the population without impacting the climate. Although the ideal technical requirements of a catalyst in providing different solutions in buildings is well understood, the realistic factors which will enable impactful deployment are exclusively associated with the cost, reliability, longevity, retrofittability, manufacturability, and ready availability of materials.
Nomenclature & Abbreviations
Btu |
British Thermal Units |
EJ |
Exajoules |
g |
gram |
h |
Hour |
kg |
Kilogram |
kJ |
Kilo Joules |
mL |
Milliliter |
MJ |
Mega Joules |
TWh |
Terawatt-hours |
CNT |
Carbon Nano Tubes |
EIA |
Energy Information Administration |
EPA |
Environment Protection Agency |
HVAC |
Heating, Ventilation, and Air Conditioning |
IAQ |
Indoor Air Quality |
IEA |
International Energy Agency |
MOF |
Metal Oxide Framework |
NIH |
National Institute of Health |
OER |
Oxygen Evolution Reaction |
ORR |
Oxygen Reduction Reaction |
Quad |
Quadrillion |
PEM |
Polymer Electrolyte Fuel Cells |
PGM |
Precious Group Metals |
RH |
Relative Humidity |
RTIL |
Room Temperature Ionic Liquids |
SAPO |
Silicoalumino Phosphate Zeolite |
SOFC |
Solid Oxide Fuel Cell |
USA |
United States of America |
VOC |
Volatile Organic Compounds |