The anti-virulence strategy is designed to prevent bacterial virulence factors produced by pathogenic bacteria from initiating and sustaining an infection.
1. Introduction
Since their serendipitous discovery by Alexander Fleming, antibiotics have been the basis in modern medicine for the treatment of bacterial diseases because of the life-saving compounds produced by Florey during World War II. However, the emergence of multidrug resistance bacteria has threatened to unravel this approach to human and animal health. The global spread of resistant microbes in all areas of disease treatment has left the antibiotic drug pipeline unable to meet demand since compounds are metabolized by voracious multi-drug resistant organisms
[1][2][3][1,2,3]. The situation begs for new strategies to combat microbial pathogens
[2][4][5][6][7][2,4,5,6,7].
A compelling, innovative, and alternative approach to antibiotic therapy is the anti-virulence or anti-infective strategy that involves targeting virulence-associated rather than survival/fitness-relevant traits in the offending pathogen
[8][9][10][11][12][13][8,9,10,11,12,13]. Thus, the “anti-infective” agent/drug interferes with the pathogen’s ability to cause disease
[14][15][14,15]. One avenue for this approach is to target bacterial toxins using this powerful method
[9][16][17][18][19][9,16,17,18,19]. This approach involves the use of patho- or virulence blockers developed specifically to bind bacterial virulence factors with high affinity, neutralizing or reducing the virulence of the offending pathogen
[17][20][21][17,20,21]. Anti-virulence compounds offer significant advantages over conventional antibiotics. Firstly, these agents are directed towards specific mechanisms in the offending pathogen that promote infection or harm host cells rather than targeting an essential growth/metabolic factor. Disarming microorganisms of their virulence properties without threatening their survival offers lower selection pressure, reducing the risk of drug-resistant mutations. Secondly, virulence-specific therapeutics avoids the collateral damage on the host microbiota associated with current antibiotics. Other possible advantages to the anti-virulence approach include limited off-target effects due to the unique mechanism of the mART toxin action and targeting a conserved motif within a family of proteins provides a potential to target multiple pathogens with a single small molecule compound. Thus, the anti-virulence approach has the potential for a paradigm shift in the development and treatment of bacterial diseases and infections. This novel approach makes pathogenic bacteria less virulent by neutralizing their weaponry, thereby giving the host’s immune system time to clear the pathogen. Additionally, the weakened pathogen is more susceptible to antibiotic therapy
[15].
Bacterial toxins of all types are a common infection tool produced by bacterial pathogens to cause disease. These factors facilitate the infection process by the pathogen and are often key elements in disease development. Compounds, known as anti-virulence agents, are designed to interfere or block the toxin machinery and associated molecular mechanisms and hold promise to thwart bacterial pathogens.
A growing collection of bacterial toxins that serve as high profile targets for anti-virulence intervention is the mono-ADP-ribosyltransferase (mART) family. Family members covalently modify specific host macromolecules as an “on—off” switch often producing a unique pathology. Notably, diphtheria toxin (DT) is a classic example of a mART toxin that is the sole cause of a disease (diphtheria) by modifying ribosomal elongation factor 2 (eEF2), terminating protein synthesis, and leading to apoptosis
[22]. The cellular targets for mART toxins are often key regulators of cell function and include (i) GTPases, (ii) actin, (iii) kinase regulators, (iv) elongation factors, and (v) RNA-recognition motifs, and even DNA (target genes)
[22][23][24][25][26][27][28][22,23,24,25,26,27,28]. We developed a data-mining strategy based on fold-recognition methods
[29][30][31][29,30,31] that unearths new mARTs as targets for disease intervention using the anti-virulence or anti-infective approach (
Figure 1).
Figure 1. Summary of mART toxin discovery pipeline. Step 1: Known mART toxin sequences are used (input) to provide several thousands (10,000–20,000) of candidate toxin sequences arising from iterative search tools such as HHblits and HHsenser. This mining strategy is analogous to a large-scale BLAST search that cater to protein homologs with low sequence identity
[32][33][32,33]. Step 2: Sequences are then subjected to pattern-based filters, including searches for the conserved mART motif, followed by predictions for transmembrane domains (unwanted), and for secretion signal peptides or other indicators of non-classical secretion. Step 3: Finally, consensus fold recognition analyses determine whether the remaining sequences may yield a mART-like fold by generating homology models. The final list contains putative mART toxin sequences for experimental testing in a yeast growth-deficiency assay to validate computer predictions
[34] [taken from Tremblay et al.
Toxins 12: 792, 2020].
2. Anti-Virulence Approach
2.1. Virtual Screens
One facet of our approach is to use relevant high-resolution crystal structures as the template models for virtual screening (
Figure 2). The PDB structure is prepared for molecular docking using appropriate software (OpenEye,
http://www.openeye.net/products/software/ (accessed on 5 May 2017); Schrodinger software suite,
https://www.schrodinger.com/ (accessed on 6 June 2018) run on a super-computer network
[35][36][68,69]. Virtual screens yield a list of potential “hits” against the anti-virulence drug target (receptor) and can produce valuable contributions in “hit-and lead-compound” discovery
[37][38][39][70,71,72] with promising results
[40][41][42][43][44][45][73,74,75,76,77,78] using iota toxin in complex with NADH (1GIQ:C2-like)
[46][47][59,64] and C3bot1 toxin (2C8A:C3-like)
[48][60]. Online compound libraries such as the ZINC Drugs NOW set of 6.5 million drug-like compounds
https://zinc12.docking.org/subsets/drugs-now (accessed on 6 June 2018) serve as a starting source of compounds for docking experiments with methods as described
[48][60]. Ultimately, only a small, manageable subset of compounds (usually less than 100) was selected for testing as inhibitors of mART toxin enzyme and cytotoxic activity
[46][48][47][59,60,64].
Figure 2. Anti-virulence development pipeline for mART toxins. mART inhibitors arise from two major sources, namely, virtual screening using a high-resolution mART structure of a toxin-inhibitor complex and “hand-picked” directed libraries (Step 1). Candidate compounds are then subjected to a Biochemical Assay (Step 2), producing a library of active, low-affinity compounds (μmol/L affinity for binding site), which are then chosen for cell-based testing (Step 3). Promising inhibitor compounds are then tested for efficacy to protect the corresponding target host cells from the mART toxin (Step 4). The most efficacious compounds (leads) are co-crystallized (Step 5) with their cognate toxin followed by lead optimization through Structure–Activity Relationships/Quantitative Structure–Activity Relationships methodology (Step 6). Combinatorial Chemistry methods are then used to prepare derivatives of the best compounds (Step 7), which are then re-tested in the Biochemical Assay (Step 8) and the cycle of development continues until higher-affinity compounds (nmol/L or greater) are developed.
2.2. Directed Libraries
We recently chose flavonoid compounds as our directed library source of small molecules
[49][79] to complement the virtual screen approach. Flavonoids are natural products produced as plant secondary metabolites and are polyphenols that feature two phenyl rings bridged with a pyran or pyranone ring (see
Table 1). Importantly, flavonoids show diverse biological activities, including antibacterial activity
[50][80], inhibitors of proteins in cells, or their membranes and membrane perturbation activity
[51][52][81,82]. Initially, a flavonoid library of 1200 compounds were sourced as potential anti-virulence agents against mARTs, and finally, 20 compounds were chosen for experimental testing based on several filter criteria, including literature reports of antimicrobial activity.
Table 1.
Chemical Inhibitors of Plx2A.
Inhibitor |
Chemical Name |
Structure |
a KD (μmol/L) |
b IC50 (μmol/L) |
Acacetin |
5,7-dihydroxy-2-(4-methoxy phenyl)-4H-chromen-4-one |
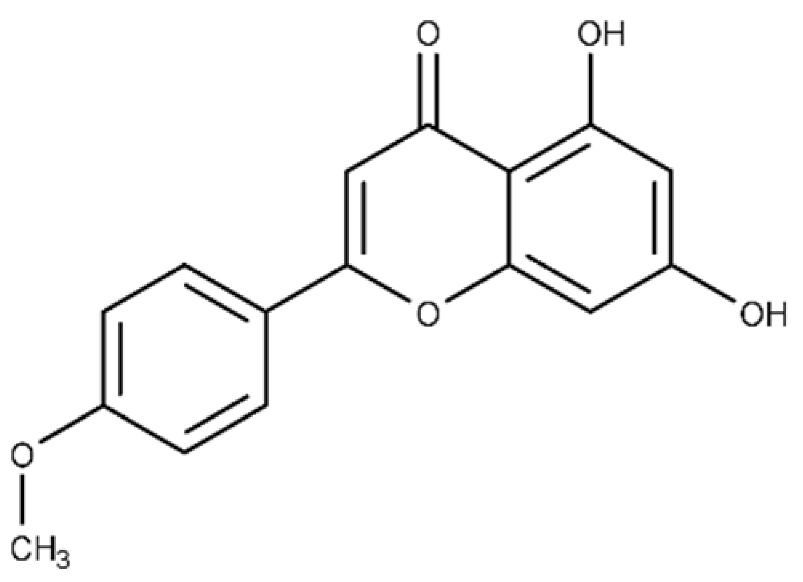 |
c ND |
28.0 ± 2.3 |
Baicalein |
5,6,7-trihydroxy-2-phenyl-4H-chromen-4-one |
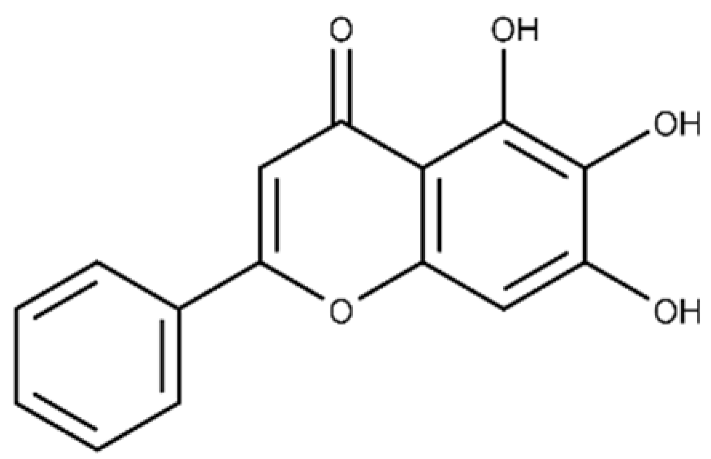 |
13.6 ± 1.5 |
10.7 ± 0.7 |
Chrysin |
5,7-dihydroxy-2-phenyl-4H-chromen-4-one |
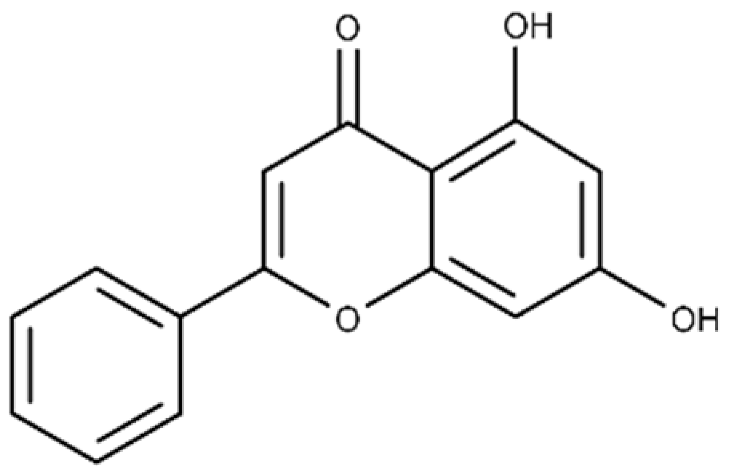 |
40.6 ± 4.7 |
49.2 ± 2.5 |
Jaceosidin |
5,7-dihydroxy-2-(4-hydroxy-3-methoxyphenyl)-6-methoxy-4H-chromen-4-one |
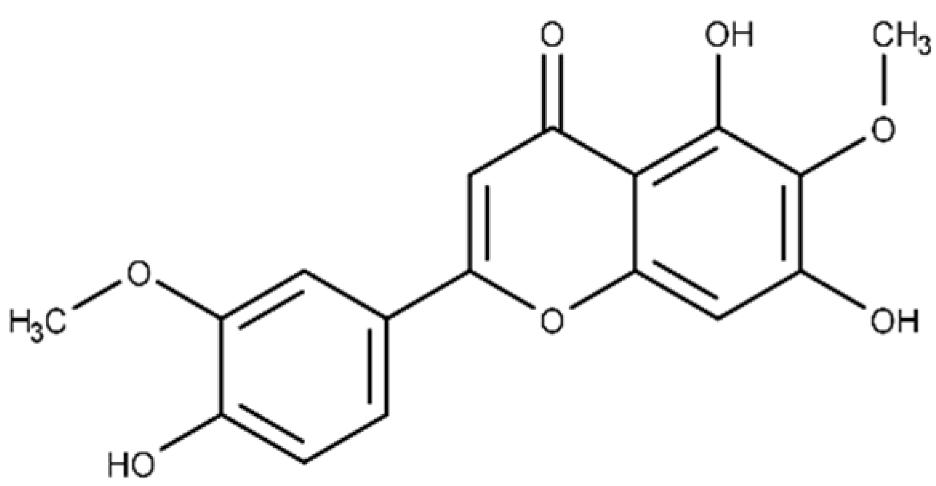 |
24.1 ± 2.9 |
73.9 ± 4.7 |
Kaempferol |
3,5,7-trihydroxy-2-(4-hydroxyphenyl)-4H-chromen-4-one |
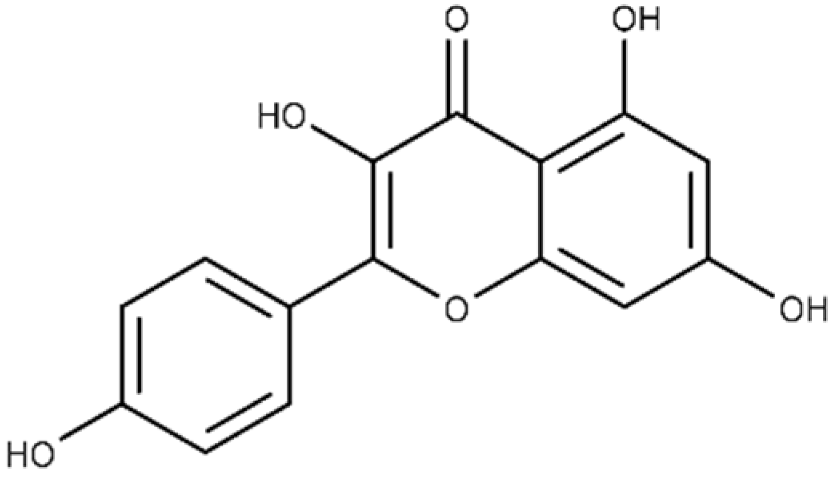 |
37.3 ± 3.6 |
79.9 ± 6.0 |
Luteolin |
2-(3,4-dihydroxyphenyl)-5,7-dihydroxy-4H-chromen-4-one |
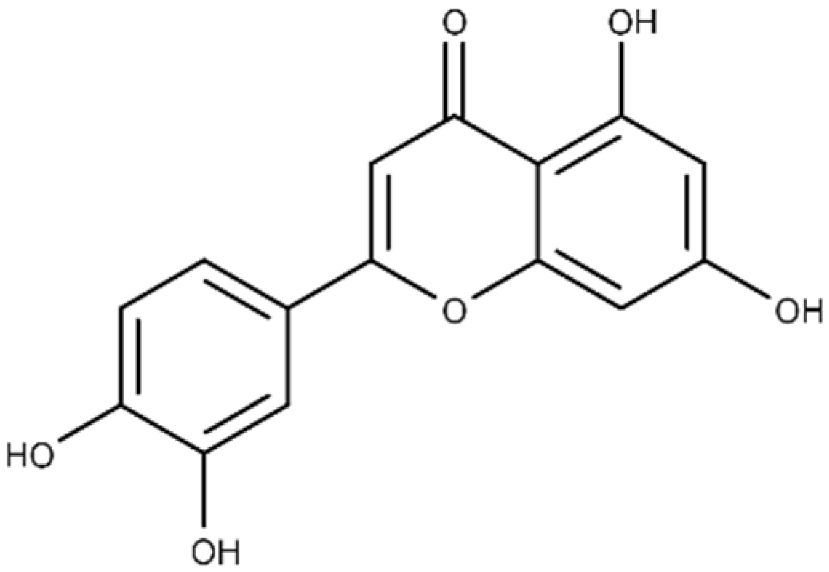 |
24.0 ± 1.5 |
68.1 ± 1.8 |
Morin |
2-(2,4-dihydroxyphenyl)-3,5,7-trihydroxy-4H-chromen-4-one |
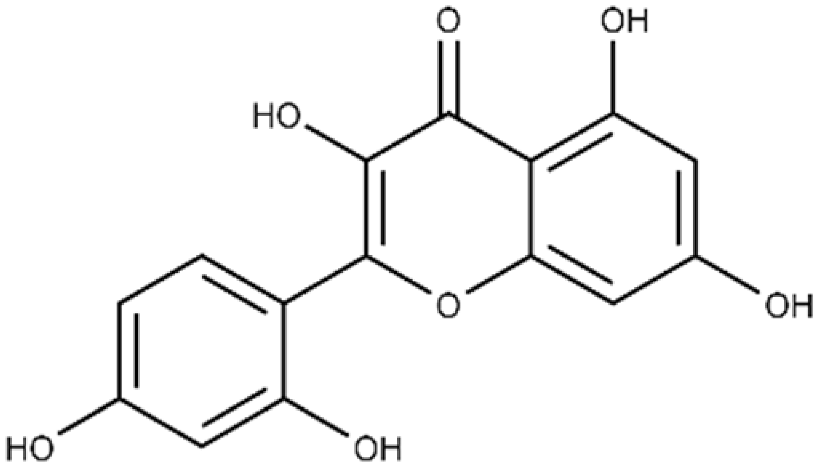 |
32.8 ± 11.7 |
109 ± 4.8 |
Quercetin |
2-(3,4-dihydroxyphenyl)-3,5,7-trihydroxy-4H-chromen-4-one |
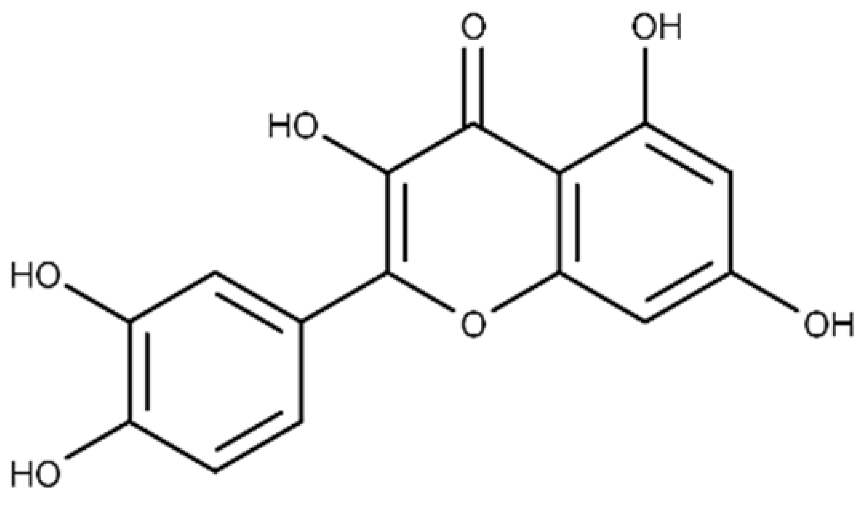 |
19.9 ± 0.4 |
24.3 ± 0.8 |
M3 |
N-{[(3R)-1-{1H-pyrazolo[3,4-d]pyrimidin-4- yl}piperidin-3-yl]methyl}methanesulfon amide |
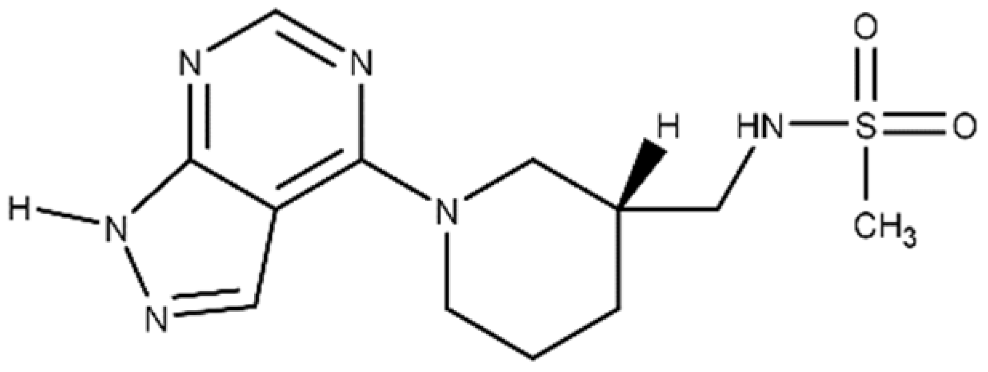 |
a The binding affinity of inhibitors to Plx2A (KD) were calculated from the binding isotherms generated from the quenching of intrinsic Trp fluorescence of the protein upon titration with the inhibitor (ligand). The values are the mean ± S.D. from three separate experiments. b The IC50 values were calculated from the fit to a dose–response curve of Plx2A GH activity against the concentration of inhibitor/flavonoid compounds to produce a directed library of 20 compounds for experimental testing against Plx2A activity [9]. c ND, not determined.
2.3. Biochemical Assay
Initial testing of the inhibitory activity against mART toxins involves an enzymatic assay, since these virulence factors are bacterial enzymes that cleave the NAD
+ substrate and transfer ADP-ribose to the macromolecule substrate. However, most mARTs also show a secondary activity known as glycohydrolase (GH) that involves direct hydrolysis of NAD
+ in the absence of a second substrate. A fluorescence-based plate-reader enzyme assay is often used to determine the inhibitor properties (IC
50, K
i values) of the selected compounds (
Figure 2). A fluorescence-based binding assay for lead compounds with the target mART is also established based on quenching of intrinsic fluorescence upon enzyme-inhibitor association to provide a measure of binding affinity (K
D)
[9].
2.4. Cell-Based Testing
Compounds with low micromolar (μmol/L) efficacy from the biochemical assay step (Figure 2) are then tested in a cell-based system that is specific for the mART-producing pathogen (Figure 2). Effective compounds are identified as those agents that provide protection of mART-intoxicated cells at low micromolar doses while not causing cellular cytotoxicity. This assay provides a direct measure of the dose–response (ED50 value) and cellular toxicity (TD50 value) of the inhibitor compounds. The best compounds are those with the highest therapeutic index (TD50/ED50) as a measure of inhibitor potential.
2.5. Crystallization of mART-Inhibitor Complexes
The co-crystal structures of mARTs with the best lead compounds are pursued to provide important structural biology insights into the location, nature, and chemistry of the inhibitor-binding sites (Figure 2). The details of the toxin-inhibitor complexes with the various mARTs provides new insights into the structural features/differences of the binding pockets for mART toxins. These structural data also provide the basis for further rationale inhibitor design to iteratively evolve/design lead hits into bona fide drug candidates.
2.6. SARs/QSARs and Combinatorial Chemistry
Rational inhibitor design and improvement involves SARs and QSARs studies to iteratively evolve lead hits into high-affinity drug candidates (
Figure 2). Lead compounds identified in virtual screens or from “directed libraries” that show promise in enzyme and mammalian cell assays are further developed with respect to binding potency and pharmacokinetic properties. These compounds are investigated initially through a combination of modeling and docking studies using the target crystal structure to see which groups are essential for target binding, while at the same time introducing water-solubilizing groups with an eye to keeping the pK
a values for compounds between 5–6. New second- and third-generation analogues can be synthesized using the unique microwave-assisted, continuous flow synthesis platform
[53][54][55][83,84,85] (
Figure 2). Conventional Lipinski parameters are used to guide analogue design
[56][86], which are obtained using Drug Discovery software. As new candidates arise from the screen efforts, they are brought through the same optimization process and the cycle continues until high-affinity compounds are achieved.
3. Anti-Virulence Agents against American Foulbrood
3.1. Paenibacillus Larvae
Honey bee (
Apis mellifera) beekeepers are faced with multiple challenges globally when maintaining bee colonies for pollination and honey production
[57][87]. American Foulbrood is a bacterial disease that afflicts the brood and is responsible for huge economic losses in the apiary
[58][59][88,89]. The disease is caused by
Paenibacillus larvae, a Gram-positive, rod-shaped bacterium that forms spores that can remain viable for decades
[60][90]. The ERIC genotypes of
P. larvae strains are named after their enterobacterial repetitive intergenic consensus sequences and exist as five strains, where only two are currently environmentally active
[61][91]. ERICI is found worldwide and is the slowest-killing phenotype, which helps to avoid the housekeeping of nurse bees, while ERICII is the most virulent species that occurs only in Europe and can infect an entire colony within a week
[62][92]. Several virulence factors produced by
P. larvae have been identified and their biology and role in pathogenicity have been reported
[58][63][88,93]. These factors include two mART toxins, Plx1 and Plx2, shown to be important ERICI virulence factors
[64][94]; Plx2A was recently characterized and crystallized
[65][61]. However, to date, only a few of the
P. larvae virulence factors have been targeted for an anti-virulence strategy
[9][65][66][9,61,95].
3.2. C3larvin Toxin
C3larvin was identified by a bioinformatics approach as a mART in the C3 toxin subgroup and putative virulence factor from
P. larvae. Cytoplasmic expression of C3larvin in a yeast model system revealed that it is highly cytotoxic
[48][60]. C3larvin catalytic variants (Q155A, E157A, Q155A/E157A) with reduced or no mART enzymatic activity, were not toxic to yeast (eukaryotic model), revealing that C3larvin was a mART toxin with enzymatically driven cytotoxicity in a eukaryotic host. C3larvin showed mART enzyme activity and labeled Asn41 on RhoA, as seen in the C3 subgroup
[48][60]. Surprisingly, C3larvin did not enter target macrophages unlike other C3 subgroup members, and it was determined that C3larvin possessed a truncated helix 1. It is now appreciated that it is only functional in a unique
P. larvae strain of the MLST sequence type 9 in ERICIII/IV
[66][95]. Two crystal structures of C3larvin revealed that it is indeed a C3 subgroup member with a shortened helix 1 (PDB:TR5; PDB:5DZQ), although the protein is not functional in bee larval assays
[67][62].
An inhibitor, M3, of C3larvin mART enzymatic activity was identified by a virtual screen
[47][64]. The methane sulfonamide derivative inhibited the enzyme with a K
i = 11 μmol/L and was the first inhibitor reported for the mART C3 subgroup
[48][60]. M3 is unusual as an inhibitor of mART toxins because it possesses an adenine ring linked to substituted piperidine. M3 was also shown to be an effective inhibitor against Vis toxin from
Vibrio splendidus and C3bot1 from
Clostridium botulinum
[47][64]. A molecular mechanics/molecular dynamics approach suggested that M3 competes with the adenine ring of the NAD
+ substrate, but verification awaits a high-resolution co-crystal structure
[48][60].