Lead-free perovskites have received remarkable attention because of their nontoxicity, low-cost fabrication, and spectacular properties including controlled bandgap, long diffusion length of charge carrier, large absorption coefficient, and high photoluminescence quantum yield. Compared with the widely investigated polycrystals, single crystals have advantages of lower trap densities, longer diffusion length of carrier, and extended absorption spectrum due to the lack of grain boundaries, which facilitates their potential in different fields including photodetectors, solar cells, X-ray detectors, light-emitting diodes, and so on.
1. Introduction
As a striking material, lead halide perovskites (APbX
3) have made unprecedented progress in various fields, such as photodetectors, solar cells, X-ray detectors, light emitting diodes, lasers, transistors, and so on
[1,2,3,4,5,6][1][2][3][4][5][6]. The merits of low-cost solution processing and remarkable optoelectronic properties, including tunable bandgap, long carrier lifetime and carrier diffusion length, large absorption coefficient, give lead halide perovskites great potential in the photovoltaic power generation field
[7,8,9][7][8][9]. Single-junction perovskite solar cells have realized a certified power conversion efficiency (PCE) of 25.5%, which is comparable to that of silicon-based solar cells
[10]. In terms of light emitting, perovskites exhibit a narrow full width at half maximum, high photoluminescence quantum yield (PLQY), and wide color gamut
[11]. Meanwhile, photodetectors, transistors, and lasers are also developed rapidly.
However, the severe toxicity and chronic degrading of lead (Pb), the aqueous solubility may cause the contamination of ground water, and the poor stability when exposed to oxygen, heat, moisture and UV light, has retarded the expanded applications of lead halide perovskites
[12,13,14][12][13][14]. Although numerous nontoxic elements have been reported as dopants, the residual Pb may still present environmental risk. Hence, the development of low-toxic lead-free perovskites is of great significant to replace the classic APbX
3 [15]. Meanwhile, in comparison with polycrystalline perovskites and low-dimension perovskites, perovskite single crystals (PSCs) show excellent optoelectronic properties due to their continuous and unbroken crystal lattices
[16], the absence of grain boundaries leads to lower trap densities, longer length for carrier diffusion, and extended absorption spectrum
[17,18,19][17][18][19]. Therefore, research of LFPSCs has promoted the enhancement of perovskite materials, and the current high-quality LFPSCs play critical roles in abundant optoelectronic devices.
LFPSCs materials are a series of compounds with a general chemical formula of A
xB
yX
z (x, y, z is up to the structural dimensionality), where A represents an organic or inorganic cation such as MA (CH
3NH
3), FA (HC(NH
2)
2, Cs, Rb, B represents a metal cation (Sn/Sb/Bi/Pd/In/Ti/Pt/Au Cu/Ag), and X represents halide anion (Cl/Br/I). In the metal halide octahedra, B-cation stay at the center of the octahedral, and six X-anions are situated at the six corners, which can grow three-dimensional (3D), two-dimensional (2D), one-dimensional (1D), or zero-dimensional (0D) crystal structures
[20,21][20][21]. The dimensionality of the perovskite crystal structures mainly depends on the size of the cations and should also fulfill the requirement of Goldschmidt tolerance factor (t),
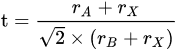
where,
rA,
rB, and
rX are the ionic radius of the A-site, B-site, and halide site, respectively. LFPSCs can be classified into four categories based on their crystal structure and the valency of the B cation: (i) divalent metal cation perovskites: ABX
3 (B is +2 oxidation state, B = Sn/Ge/Yb) or layered perovskites A
2A′
n−1B
nX
3n+1; (A′ = long chained organic cations that do not fit in the [BX
6]
4− cavity); (ii) trivalent metal cation perovskites: A
3B
2×9 (B is +3 oxidation state, B = Sb/Bi); (iii) tetravalent metal cation perovskites: A
2BX
6 (B is +4 oxidation state) (B = Sn/Ge/Pd/Pt); and (iv) double perovskites: A
2BB′X
6 (B is +1 while B′ is +3 oxidation states) (B is Au/Ag/ACu; B′ is In/Bi/Sb)
[12,22][12][22].
2. Various Systems of Pb-Free Single Crystal
In general, lead halide perovskites possess a universal chemical formula of APbX3, where A represents an organic/inorganic cation including Cs+, methylammonium (MA), formamidinium (FA) or their mixture, and X represents a halide anion which consists of Cl−, Br−, I−, or their mixture. In terms of structure, Pb2+ cations are separated by six neighbor X-site anions to bulid Pb-X octahedrons, which corner-share with each other to constitute the main frame and A+ intercalates the voids [24][23]. The replacement of Pb2+ with lead-free ions results in both the deformation in nanoscale structure and the conversion of properties because of the differences in chemical valence and ion size [23][24]. Therefore, LFPSCs exhibit plenty of novelty and diversity.
2.1. Sn Based Halide Perovskites
Attributed to the same valence and similar properties with Pb
2+, Sn
2+ is seen as a crucial candidate to form lead-free perovskites without the sacrifice of the excellent performance. The first Sn-based halide perovskite single crystals were synthesized in 1974 for CsSnX
3 [25]. In 2012, Chung et al. prepared CsSnI
3single crystals using a modified vertical Bridgman technique with refined crystal structure and optical properties
[26]. Due to the crystal structure transformation from α-phase to γ-phase during the preparation of CsSnI
3, the crystal configuration of CsSnI
3 is not cubic symmetry, but the stable γ-phase CsSnI
3 SC (direct bandgap =1.3 eV) at room temperature, showing a p-type semiconductor behavior with a carrier concentration and a hole mobility of ≈10
17 cm
−3 and ≈585 cm
2 V
−1 s
−1, respectively. A similar result has been reported in FASnI
3 perovskite single crystals by Kahmann et al.
[27].
2.2. Bi/Sb Based Halide Perovskites
Trivalent ions such as Bi
3+ and Sb
3+ are also considered as the alternatives for LFPSCs. Generally, B
3+ can form a perovskite-like derivate—A
3B
2X
9, with 0D or 2D crystal structures. The initial exploration started by Lehner and co-workers in 2015
[30][28]. They found A and X atoms are closest-packed while B atoms occupy 2/3 voids of the octahedral X
6, and the crystal structures of A
3B
2X
9 can be classified into two typical types: cubic close-packing and hexagonal close-packing of A and X atoms. Changes of A cation lead to significant differences in structural configurations and properties. To be specific, Cs
3Bi
2I
9 single crystal tends to form a 0D configuration attributed to the isolated [Bi
2X
9] structures, which is resulted from the face-sharing [BiX
6] octahedron. By contrast, K
3Bi
2I
9 and Rb
3Bi
2I
9 tend to generate layer-like 2D structures. Another popular trivalent ion is Sb
3+. McCall and co-workers synthesized Cs
3Bi
2I
9 and Rb
3Bi
2I
9 SCs by the Bridgman method and characterized their crystal structures by SCs X-ray diffraction, showing [SbI
6] octahedrons and isolating alkali ions for both of them. For optoelectronic properties, they display broad PL emission from 1.75 to 2.05 eV with two peaks located at 1.96 and 1.92 eV, respectively
[31][29]. Besides that, these scholars have made a further investigation on other derivatives, after optimizing the synthesis of single crystal, they implemented the photo-response to observe visible laser emission of Cs
3Bi
2I
9 for the first time. All SCs showed ambipolar response to Am α-particles irradiation with spectra for both electron and hole collection configurations, and it is worth noting that Cs
3Bi
2I
9 and Cs
3Sb
2I
9 showed a superb potential for radiation detection
[32][30].
2.3. Other Metals Based Perovskites
Researchers have also developed some other metal-based PSCs, such as from indium, copper. In the work of Zhou et al., they employed a slow-cooling crystal growth approach by blending CsBr and InBr
3 in HBr at 130 °C for 0.5 h
[33][31]. After cooling down to room temperature, 0D Cs
2InBr
5:H
2O was obtained with a size of around 2 mm. It shows a 0D orthorhombic crystal structure where the [InBr
5O]
4− octahedrons were separated by two Cs
+-cations. It also displays a bright red luminescence peak at 695 nm with a PLQY of 33% under excitation of 365 nm. Other 0D (C
4H
14N
2)
2InBr
10 PSCs were synthesized within several minutes by adding InBr
3 solution (dissolved in HBr acid) into a mixture of diethylamine and HBr at 0 °C
[34][32]. The In-Br polyhedrons were separated by the (C
4H
14N
2)
2+-cations to produce a 0-D perovskite-like structure. It exhibits an abrupt absorption from 350 to 600 nm and a broad band emission from 500 nm and near-infrared region attributes to the structural distortion of [InBr
6]
3- octahedral units leading to the formation of self-trapped exciton (STE) states, confirmed also by computational study.
2.4. Halide Double Perovskites
Apart from single B-site ions-based lead-free perovskite, double perovskites with a formula of A
2B′B″X
6 have been investigated due to their excellent performance
[37,38,39][33][34][35]. Pan et al. used a solution-process approach to obtain double perovskite Cs
2AgBiBr
6 single crystals, where centers of the metal bromide octahedron are occupied by alternate Bi
3+ and Ag
+. They proposed the presence of cations disorder during the growth process, resulting in the destroyed symmetry of double perovskite. Thermal annealing and surface treatment could eliminate these defects and improve the crystal resistivity effectively
[40][36]. After that, numerous researchers have accomplished research on Cs
2AgBiBr
6 [41[37][38][39][40],
42,43,44], in the work of Zhang et al., the resistivity of the Cs
2AgBiBr
6 was larger than 10
10 Ω cm, the Fermi level was estimated to be 0.788 eV above the valence band and the two near bandgap energies were 1.917 eV and 2.054 eV, respectively
[41][37]. Keshavarz and co-workers employed alkali substitution to tune the structures and properties of Cs
2AgBiBr
6 double perovskites. The fundamental lifetime of carrier recombination at room temperature attained a three-fold increase with the band gap remaining unchanged
[44][40]. Furthermore, Yin et al. synthesized Cs
2AgIn
xFe
1−xCl
6 (0 < x < 1) perovskite SCs employing a simple hydrothermal method, which exhibited a broadband absorbance from 450 to 800 nm and a huge enhancement of PLQY
[45][41].
3. Applications
LFPSCs possess numerous fascinating optoelectronic properties in practical applications, as shown in Figure 1. Even if there is still a certain gap between lead-free and lead-based PSCs, several applications of LFPSCs have attracted attention recently. Herein, the reported achievements of applications using LFPSCs, such as photodetectors, solar cells, X-ray detectors, light emitting diodes, and other applications (Figure 2).
Figure 1. Schematic diagrams of synthesis methods of LFPSCs. (a) Bridgeman method. (b) Cooling-induced crystallization method. (c) Inverse temperature crystallization. (d–h) Typical crystal structures of LFPSCs. Reprinted (adapted) with permission from Reference 12. Copyright 2020 Elsevier Ltd. Reprinted (adapted) with permission from Reference [22]. Copyright 2021 American Chemical Society.
Figure 2. Illustration of various remarkable properties of LFPSCs in practical applications, including photodetectors, X-ray detectors, and light-emitting diodes. Reprinted (adapted) with permission from Reference
[23][24]. Copyright 2021 WILEY-VCH Verlag GmbH & Co. KGaA, Weinheim.
3.1. Photodetectors
Photodetectors capture optical signals and convert them into electrical signals instantaneously, which have been widely employed in abundant fields. The key factors of excellent photodetectors can be summarized as fast responding speed, high photocurrent intensity, and low detectivity.
Liu et al. reported a blue light photodetector with a structure of Si/SiO2/Cs3Sb2Br9/Au, the device possessed low dark current (2.4 × 10−12 A) and impressive photocurrent (3.1 × 10−8 A) at a bias of 6 V under dark condition and illuminated by 480 nm light, the response and recovery time was 0.2 ms and 3 ms respectively [49][42]. Compared with other lead-free perovskite-based photodetectors, Zheng et al. fabricated a nanoflake photodetector demonstrating a response speed of 24/48 ms [50][43].
3.2. Solar Cells
He et al. fabricated the device using synthesized FASnI3 single crystals as precursors, which possessed high purity, low defect density, and excellent stability in the air [59][44]. The authors demonstrated that re-dissolved single crystals forming solution effectively prevents the oxidation of Sn2+ by reducing impurities and moisture. The single crystal precursors-based films showed smooth morphology and exhibited larger and more uniform grains than conventional films. The PCE of device was 8.9% and 5.5% for spin-coated solar cells and large-scale printed cells, respectively. In addition, FASnI3 single crystal precursors-based devices retained a higher percentage of initial PCE than conventional devices. The precise controlling of crystallization to obtain near-single-crystalline film is also a viable approach to achieve higher performance.
3.3. X-ray Detectors
X-ray detection plays an important role for scientific study, medical diagnosis, and industrial inspection
[12,70][12][45]. LFPSCs can be good candidates for X-ray detection because of some unique properties including large X-ray attenuation coefficients; a suitable bandgap (1.5 to 5.0 eV); highly crystalline with lower trap density; large bulk resistivity with less ion migration; high sensitivity and stability; and low toxicity
[71,72][46][47]. The detection of X-ray has been of great importance due to the wide applications of X-ray in various fields, and some relevant works are summarized in
Table 41 on this topic.
3.4. Light-Emitting Diodes
Table 1. X-ray detection parameters of LFPSCs based devices.
LEPSCs |
μτ Product (cm2V−1) |
Sensitivity (μC·Gyair−1·cm−2) |
Detection limit (nGyairs−1) |
Ref. |
Cs2AgBiBr6 |
6.3 × 10−3 |
316.8 |
59.7 |
[36] |
Cs2AgBiBr6 |
5.95 × 10−3 |
1974 |
226.2 |
[48] |
MA3Bi2I9 |
NA |
1947 |
83 |
[49] |
Cs3Bi2I9 |
7.97 × 10−4 |
1652.3 |
130 |
[50] |
(BA)2CsAgBiBr7 |
1.21 × 10−3 |
4.2 |
NA |
[51] |
(H2MDAP)BiI5 |
NA |
1.0 |
NA |
[52] |
3.4. Light-Emitting Diodes
Another important optoelectronic application of perovskites is light-emitting diodes (LED) because of their PLQY, tunable band gap, and facile solution preparation. For LFPSCs, low-dimensional halide perovskites have attracted remarkable attention for their spectacular photoluminescence properties and chemical stability, also, the doping strategies have been widely adopted to enable or balance multiple emission centers
[83,84,85][53][54][55]. In 2019, a (C
8NH
12)
4Bi
0.57Sb
0.43Br
7·H
2O SCs was synthesized and showed ultra-broadband emission spectrum between 400 and 850 nm, with a PLQY value increased from 0.7% ((C
8NH
12)
4BiBr
7·H
2O) to 4.5%
[83][53].
3.5. Humidity Sensor and Field-Effect Transistors
Besides the above-mentioned applications, Pb-free PSCs were also employed into other promising applications. Zhou et al. prepared a Pb-free 0D Cs
2InBr
5·H
2O PSC with a broad red luminescence centered at 695 nm and a high PLQY up to 33%, resulting from the deformations of charge carriers via STE states
[33][31]. It exhibits different emission in a moisture-containing condition with good structural- and photo-stability. A PL humidity sensor was fabricated based on switchable dual emission corresponding to the hydrated and dehydrated states, showing good recyclability and fast response time. This pioneering work establishes a foothold for the utilization of Pb-free perovskite in humidity detection and also demonstrates the advantages of exploring novel applications for these Pb-free perovskite materials.
4. Challenges and Prospects
The current challenges and prospects of LFPSCs are summarized as follows:
(1) The performance of LFPSCs extremely depends on the synthesis methods, hence the exploration of controllable and reliable synthesis to yield stable and high-quality single crystals is necessary to be focused on, with economic and environmental factors under consideration. Moreover, the precise control of thickness and size could be effective approaches to enhance the performance of different devices.
(2) The doping strategies, employed to improve properties, are universal in the research of LFPSCs, but the specific enhancement mechanism has not been thoroughly studied. The intensive study of dopants contributes to obtaining tunable and enhanced single crystals. Most LFPSCs possess wide bandgap which exhibited a narrow range absorption of the solar spectrum. It is important to design more materials with favorable bandgaps and study the structure-property correlation systematically by tuning their bandgaps through halide exchange.
(3) The reproducibility of LFPSCs based devices remains a huge challenge. Almost all the reported devices are fabricated based on lab-scale. They demonstrated batch-to-batch variations, and most of their results cannot be reproduced. The properties of the synthesized materials highly depended on the operator and condition for processing, storage.
(4) The large thickness of LFPSC along the direction of carrier transportation may lead to low current density, while the fabricating technologies of devices also suppress the PCE of solar cells.
(5) Stability of LEFPSs is another important issue. Therefore, huge efforts should be devoted to improve crystal stability as well as maintain their excellent photophysical and chemical properties.