1. Kynurenine pathway
The kynurenine pathway (KP) is the major catabolic route of the essential amino acid, tryptophan (Trp), which generates variety of bioactive metabolites (derived from kynurenine) and an important enzyme cofactor, NAD+ (
Figure 1). The activity of one of three catabolic enzymes, tryptophan 2,3-dioxygenase (TDO) - expressed in a liver - and two isoforms of indoleamine 2,3-dioxygenase (IDO1 and IDO2) - expressed in various cell types - lead to KP activation in different tissues
[1][2]. During inflammation, Trp depletion via the kynurenine pathway is greatly accelerated in response to interferon-γ (IFN-γ)
[3]. The process is initiated by the activity of IDO1 enzyme and might take place in different cells of the human body like macrophages, dendritic, and tumor cells
[3][4]. KP starts from the generation of formylkynurenine, which is rapidly converted to kynurenine (Kyn) - the precursor for other catabolites including 3-hydroxykynurenine (3HKyn), kynurenic acid (Kyna), 3-hydroxyanthranilic acid (3HAA), anthranilic acid (AA), xanthurenic acid (XA), and quinolinic acid (QA)
[5]. Some Trp metabolites bind to receptors expressed by immune cells to promote tolerogenic responses
[4]. For instance, Kyn is an immunomodulatory molecule that inhibits T-cell proliferation, reduces the activity of natural killer cells and dendritic cells, and promotes the differentiation of regulatory T-cells (Tregs)
[6][7]. Kyna promotes monocyte extravasation and controls cytokine release
[6]. Furthermore, Kyn and Kyna are both ligands of aryl hydrocarbon receptor (AhR), which is involved in multiple physiological functions, tumor invasion and/or migration
[8][9]. Kyna also acts as an antagonist of a glutamate receptor and the alpha-7-nicotinic acetylcholine receptor, as well as an agonist of G-protein coupled GPR35 receptor
[10]. 3HAA induces apoptosis in monocyte/macrophage cell lines
[11]; 3HKyn suppresses CD4+ T-cell proliferation, induces Tregs development, and prolongs corneal allograft survival
[12]. QA exerts neurotoxic effects via at least nine different mechanisms
[13]. Photochemically active XA leads to apoptosis of the epithelial cells in lense
[14] and shows diabetogenic properties
[5]. On the other hand, KP metabolites are recognized for their beneficial properties including antioxidant (3HKyn, 3HAA, AA, XA, Kyna),
[5][15][16][17], neuroprotective (Kyna)
[15], vasorelaxant (XA)
[17], and anti-inflammatory (Kyna) factors
[5].
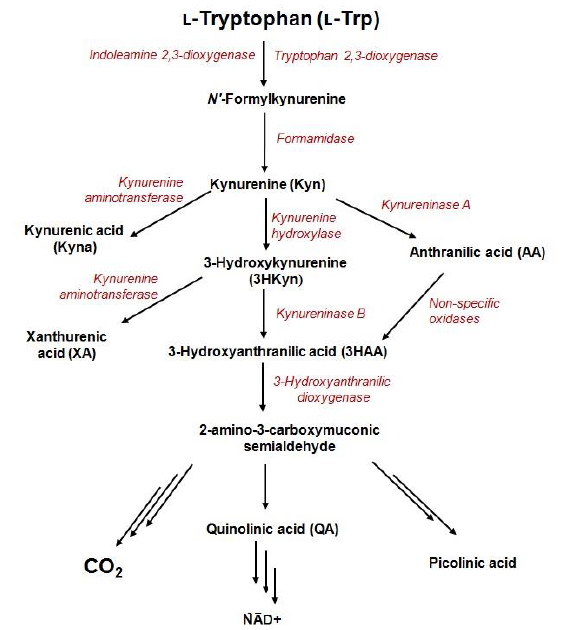
Figure 1. Kynurenine pathway.
Exploration of the role of tryptophan metabolism provides novel diagnostic and treatment opportunities, however, it requires reliable methods for quantification of its metabolites in a variety of biological samples.
2. Developments in Electrochemical Sensors for the Determination of Kynurenine Pathway Metabolites
The basis of the electrochemical analysis is the reaction that occurs on the surface of a working electrode. Thus, the selection of the working electrode is the crucial preliminary step for successful electrochemical analysis
[18]. Oxidation signals of KP metabolites (Kyn, Kyna, 3HKyn, 3HAA, AA, and XA) can be measured at a bare glassy carbon electrode (GCE) by differential pulse voltammetry (DPV). Glassy carbon is widely used as an electrode material because of its chemical stability, broad potential window, and low cost
[18].
Table 1 collects parameters on the oxidation potentials (E
ox) of different KP metabolites estimated in phosphate buffered saline (PBS) at pH 7.7. Under experimental conditions, QA signal was not detected. One can assume that the QA oxidation peak appears at a high oxidation potential and is difficult to measure at a bare GCE. Owing to high E
ox, electrochemical determination of Kyna can be also problematic. It has been explained by Kato et al.
[19]. To note, in DPV at GCE in typical supporting electrolytes for potentials >+1.2 V, the background current increases and baseline drift is observed. It significantly decreases the S/N and makes it difficult to measure signals from analytes. A boron-doped diamond electrode (BDDE) exhibits wider electrochemical windows for aqueous media
[20] and a lower background current magnitude than GCE
[21], making BDDE more suitable for Kyna detection. All the above suggest that new electrode materials with high electrocatalytic activity or other solutions should be exploited to allow direct electrochemical detection of QA and Kyna at low, physiologically relevant levels. Addressing these limitations, QA and Kyna are currently preferably measured in biological samples by LC-MS-based methods
[22][23][24]. It is noteworthy that QA is difficult to quantify by LC-MS or LC-MS/MS because of short retention time, low
m/z, and poor ionization efficiency in the MS source
[25]. The determination of this metabolite using HPLC-UV can also be problematic
[26].
So far, only one paper reports indirect electrochemical detection of QA in human serum using a biosensor modified with quinolinate phosphoribosyl transferase (QPRT) enzyme
[27]. In this approach of a sensor design, the glass support coated with indium tin oxide (ITO) has been modified with reduced graphene oxide and QPRT enzyme. The formation of an enzyme-ligand complex (QA-QPRT) at the electrode surface leads to electron release that is monitored through DPV. This method exhibited linearity in the concentration range from 6.5 μM to 65 mM, and was tested in a diluted serum matrix. However, QA concentration in serum is frequently <1.0 μM and, together with a need for sample dilution before analysis, represents a limitation for the applicability of this sensor. Admittedly, dilution of the sample minimizes the negative influence of the matrix components on the sensitivity, however the concentration of the analyte also decreases.
Direct, simultaneous determination of Kyna and XA
[28] or 3HAA and AA
[16] at a bare GCE using DPV has already been applied to study the antioxidant activities of their coordination complexes with Fe. These metabolites display good resolution of their voltammetric signals and can be measured simultaneously (
Table 1). However, the study was conducted only in phosphate buffer (0.1 M, pH 7.4) in the absence of a biological matrix and LODs were not calculated. Thus, it is difficult to assess the usefulness of these protocols for quantitative analysis of Kyna, XA, 3HAA, and AA in biological samples.
It seems that bare electrodes are not an ideal choice to conduct direct and selective electrochemical determinations of KP metabolites at low physiological concentration levels. Furthermore, considering the relatively high E
ox of Kyna at bare carbon-based electrodes and possible interferences from sample matrix components, electrochemical determination of Kyna can be difficult or even impossible. Modification/functionalization of the electrode surface is a well-recognized, effective strategy for the improvement of both sensitivity and selectivity of electrochemical measurements
[29] making it useful for quantification of Kyna and other KP metabolites. The functionalization of the multi-electrode platform composed of a BSA-pseudo Kyna molecule that in combination with the selective Kyna antibody and subsequent interaction with the anti-IgG-HRP antibody (secondary-Ab) was used to fabricate a sensor. It allowed for Kyna determination at low nM concentrations by chronoamperometry or electrochemical impedance spectroscopy
[30]. However, the biosensor is not inert to the sample matrix. Sample dilution is recommended to minimize nonspecific adsorption of serum components on the surface of the electrode.
Advantages of the GCE and BDDE surface modification have also been emphasized during the design of voltammetric sensors for Kyn [31][32]. Electrochemical deposition of Bi film onto the BDDE surface presents an easy and rapid way to improve the sensor sensitivity toward Kyn measured by DPV and it reaches a low LOD (30 nM) [31]. The coating of a GCE surface with a thin layer of Nafion polymer allows for detection of lower contents of Kyn, as the cationic form of this molecule can be pre-concentrated onto the electrode surface before the stripping step [32]. The Nafion layer can be formed by a drop-coating method (without the need for sophisticated apparatus) and easily removed by polishing using alumina slurries. Furthermore, Kyn can be effectively accumulated onto the Nafion-coated GCE at the potential of +0.5 V in 0.1 M H2SO4, before being stripped by scanning potential toward more positive values [32]. This strategy allows for working with diluted samples and decreases some interferences delivered from the sample matrix components. The applicability of Bi film-modified and Nafion-coated sensors was confirmed for the analysis of material derived from cultures of human cancer cells. Karami et al. have also developed the sensor for Kyn quantification in culture medium collected from cancer cells, but applying a multi-stage modification of the surface of the screen-printed gold electrodes (AuSPEs) [33]. The protocol for the modification of AuSPEs’ surface includes the deposition of carboxylated multiwall carbon nanotubes and immobilization of monoclonal antibody (mAb) specific to Kyn.
Other researchers proposed the application of molecularly imprinted polymers (MIPs) to allow for the selective electrochemical measurements of 3HAA in urine
[34]. To fabricate a sensor for 3HAA, the AuSPE was coated with a solution of poly(ethyleneco-vinylalcohol) containing the template molecule, released further by a surfactant. The sensor works with integrated potentiostat in flow injection mode and allows for 3HAA determination at low nanomolar levels.
3. Interferences
Biological samples are complex media since they contain multiple organic and inorganic components, along with the presence of trace amounts of a target molecule
[35]. The overlapping signals from electro-active compounds that have relatively close oxidation potentials pose one of the major problems during electrochemical analysis of a real sample. The careful selection of the electrode support and the measurement conditions allows for improvement of selectivity and sensitivity of electrochemical measurements
[31]. Regarding the electrochemical determination of KP metabolites, special attention should be paid to the following issues:
- Influence of Trp on Kyn and AA signals;
- Overlapping signals from 3HKyn and 3HAA;
- Interferences delivered by tryptophan metabolites formed in other pathways;
- Effect of amino acids;
- Interferences from uric acid, ascorbic acid, and dopamine.