Integrated microfluidic platforms (IMPs) refer to diagnostic or analytic platforms that utilize, in part, a microfluidic-based system to facilitate the combination of several different steps of medical diagnoses toward full or partial automation. The integration of microfluidics in novel diagnostic technologies takes advantage of micrometric-scale fluids behaving very differently than fluids at volumes seen in our everyday lives. The two main types of currently existing microfluidic systems are continuous-flow and droplet-based systems that can both be further classified based on their respective materials and detection methods.
1. Introduction
Droplet microfluidic systems produce a large quantity of distinct fluid microenvironments that are suspended in a separate immiscible phase, with each acting as independent miniature bioreactors
[1]. In droplet microfluidics, individual droplet microenvironments of discrete volumes are formed through the controlled, segmented flow of various reagent-containing fluids and encapsulating them in an immiscible medium to form the individual droplets
[1]. This contrasts with continuous-flow microfluidic systems, which utilize steady-state liquid flow through narrow channels or porous media to introduce necessary samples to reagents and form one homogenous mixture. Through microfluidic integration, either of these main types of microfluidic systems can offer their own respective advantages and disadvantages to a previously non-integrated diagnostic method.
Both types of microfluidic systems can be leveraged as a complementary component to existing diagnostic tools to attain complete or partial automation, multiplexing and high throughput
[2][3]. These medical diagnosis steps generally include sample preparation, sample collection and labeling, signal reading and data analysis.
Figure 1 shows a schematic workup of a continuous-flow integrated microfluidic device used for pathogen diagnosis using a PCR-based technique
[4]. Continuous-flow microfluidic devices can generally be further classified through the shape of their microchannels. The four main sub-types of continuous-flow microfluidic devices include serpentine, spiral, oscillating-flow and straight microchannels
[5]. As shown in
Figure 1, the need for multiple trained operators, external reagent preparation and laboratory-scale equipment was removed in this serpentine continuous-flow microfluidic device
[4]. In addition, this integrated continuous-flow microfluidic device is fully automated and can process the data collected from the sample in real time. The network of microchannels can be designed and tailored to fit the needs of the desired analysis and can offer several different advantages compared to conventionally sized systems. Droplet microfluidics, the second main type of microfluidic system, can offer similar improvements to modularity and automation to a continuous-flow system compared to non-integrated microfluidic systems.
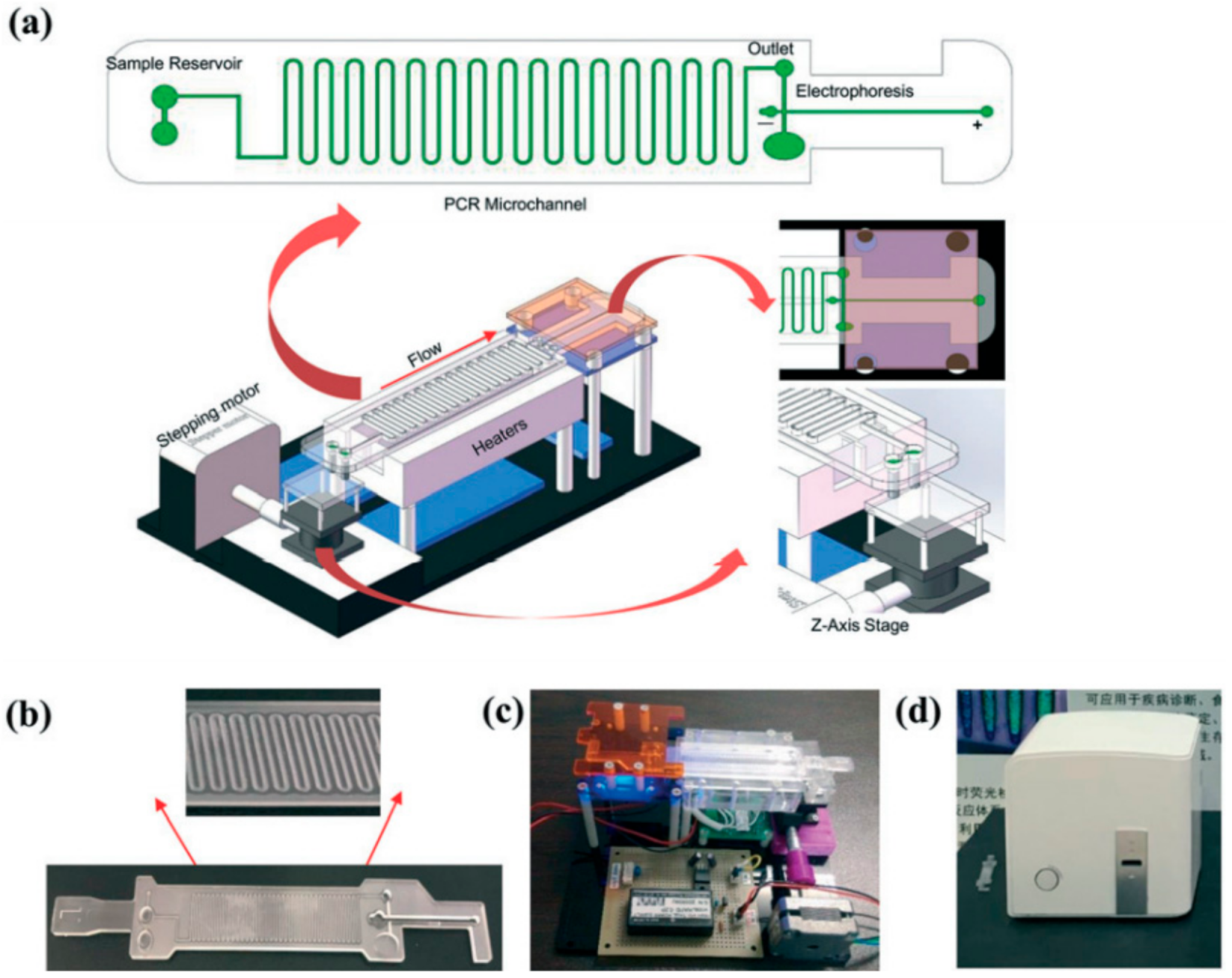
Figure 1. A schematic workup of an integrated microfluidic device that is used for pathogen diagnosis using a PCR-based technique. (
a) A microfluidic device that will be incorporated with other electronic components to automate the diagnostic process. (
b) The physical microfluidic chip is built based on the initial design. (
c) The prototype of the integrated microfluidic device. (
d) The final “all-in-one” version of the integrated microfluidic device. Reprinted with permission from ref.
[4]. Copyright 2019 Royal Society of Chemistry.
As shown in
Figure 2, droplet microfluidics can be applied to a variety of different operational mechanisms; thus, they offer their own type of subclassifications
[1]. In the case of droplet microfluidics, as shown in
Figure 2, the three main sub-types of droplet microfluidic devices are ultrahigh throughput, digital and controlled droplet microfluidics
[1].
Figure 2 offers a useful visual representation of the versatility that is achieved with a droplet microfluidic platform and effectively demonstrates the mechanistic goals of each sub-type
[1]. However, droplet microfluidics is often tailored toward extremely high throughput and single-cell tasks, which cannot be readily achieved through their continuous-flow counterpart. Moreover, droplet microfluidic platforms are more capable of high-throughput assays through the production of a high number of contained microenvironments, which is a feat that is extremely difficult to achieve through continuous-phase microfluidic systems.
Figure 2. A visual summary of several key forms of droplet microfluidics. Reprinted with permission from ref.
[1]. Copyright 2017 Royal Society of Chemistry.
Both continuous-flow and droplet microfluidics can be further categorized and classified by either the type of material used to build the device or by the mechanism of detection
[6]. Based on the type of material used, the classification of microfluidic devices typically falls under one of four kinds: glass, silicon, polymer and paper
[7]. Each of these types of microfluidic devices can be fabricated in different ways, at different costs and each presents its own unique combination of advantages and disadvantages
[6]. Depending on the goal of the microfluidic device being integrated into a diagnostic device, one can select the material of microfluidic device that best suits their needs. In addition to the classification by material, microfluidic devices can be classified by the detection mechanism, such as PCR, nanoparticles, antibodies, aptamers, molecular diagnostics and smartphone-based diagnostics. This review mostly focuses on the assessment of PCR-based and immunoassay-based diagnostic techniques that demonstrate potential for the detection and quantification of infectious pathogens through the integration of microfluidics.
2. Clinical Translation of Integrated Microfluidic Devices for Detection and Quantification of SARS-CoV-2
Current microfluidic devices for the detection and quantification of SARS-CoV-2 in patient samples hold great promise for future on-site diagnostic use. The increase in detection speed, specificity and sensitivity achieved through microfluidic integration largely improve the potential use of these devices in low-resource settings where access to diagnostic tests is severely limited [8][9]. However, many of these techniques are limited by the cost of fabrication in mass production, as well as in their robustness and durability outside of laboratory settings. The hope is that with modifications, these different devices and techniques might be able to eventually achieve microfluidic integration that can be used on-site and reliably diagnose patients infected with SARS-CoV-2.
In Table 12 , we present several notable characteristics of four of the most promising SARS-CoV-2 detection technologies currently available. The listed technologies have shown significant advancements in their potential for clinical translation through the integration of microfluidics. One example of note is the work of Qu et al. on microflow cytometry [10]. Despite not currently existing on a mobile platform, the low detection limit, extremely short total assay time and the relatively low cost of this technology demonstrates the effectiveness of novel microfluidic-based integrated diagnostic devices for detecting SARS-CoV-2 in extremely small sample volumes [10]. Although many of the conventional detection methods, such as immunoassay and RT-PCR, have comparable specificity, sensitivity and short turnaround time as a result of microfluidic integration, it does not compare well to other more novel methods. Detection methods such as microflow cytometry and nanoparticle-based detection may offer more of an inherent advantage to SARS-CoV-2 diagnosis solely based on factors such as detection limit, sample volume and quantitative capabilities; however, as previously mentioned, an optimal on-site integrated microfluidic diagnostic device for SARS-CoV-2 detection must adhere to the ASSURED standards set out by the WHO and the seven pillars of microfluidic integration to the best of their ability [11]. Therefore, microfluidic integration appears to hold the most promise in facilitating the clinical translation of our currently existing technology toward a cost-effective, rapid and selective diagnostic tool for SARS -CoV-2 that will be more readily accessible to people from low-resource areas, as well as other parts of the world.
Table 12. Comparison of several recent advancements in SARS-CoV-2 detection methods and their future on-site diagnostic potential for SARS-CoV-2.
|
Immunoassay |
RT-PCR |
Nanoparticle |
Microflow Cytometry |
Reagent |
| Consumption |
10 µg (in tube) |
20 µL (in tube) |
Negligible |
50 µL (in tube) |
Target of Detection |
IgG, IgA, IgM |
N gene, E gene |
Gold-spiked |
IgM, IgG |
Limit of Detection |
0.15 mg/L |
1-10 copy per µL |
0.08 mg/L |
0.06-0.10 mg/L |
Total Assay Time |
1 h |
2 h |
2–5 h |
30 min |
Sample Volume |
20 µL |
120 µL |
1 µL |
10 µL |
Assay Control |
Automated |
Manual |
Manual |
Automated |
Cost per Test |
~ 6 (USD) |
~ 4 (USD) |
~ 10 (USD) |
~ 5 (USD) |
Quantitative |
No |
Yes |
Yes |
Yes |
Mobile |
Yes |
Yes |
No |
No |
Stage-Wise Implementation of Microfluidic SARS-CoV-2 Detection
The use of IMPs in SARS-CoV-2 detection can be divided into two categories, with different target populations for each. While a single type of device operating on a “one-size-fits-all” principle could suffice for all SARS-CoV-2 detection, having this differentiation would increase the testing efficiency and the distribution of resources between the two. There are minute differences between healthy, symptomatic, asymptomatic and pre-symptomatic populations that would warrant changes in the device, testing mechanism or sample preparation that are better suited for the intended goal of the diagnostic test
[14].
The first type is general testing, which would be used in the screening of healthy people and those who are infected but are pre-symptomatic or asymptomatic. Currently, with over 500,000 SARS-CoV-2 cases confirmed every day and testing positive rates between 0 and 50%, a conservative estimate for the number of tests required each day is well into the millions
[15][16]. Having a low cost per test is highly beneficial to ensure the accessibility of these tests, especially for low-income communities. As discussed before, this can be achieved through reducing material costs, such as using a μPAD fabricated with wax printing. Next, a shortened turnaround time for a screening test is also favored. There are two types of SARS-CoV-2 tests available in Canada, with the Abbott Panbio™ COVID-19 Rapid Antigen Self-Test being in closer relation to general screening tests. This qualitative POC device tests for SARS-CoV-2 from a nasopharyngeal swab in a lateral flow assay and can be completed within 15 min
[17][18]. Since a qualitative answer could suffice in a general screening test, an IMP developed for general SARS-CoV-2 screening may report its results qualitatively or through simple quantitative colorimetric results using continuous flow microfluidics to conserve time and resources. A turnaround time of 15 minutes is necessary, as longer turnaround times would delay self-isolation and increase the risk of transmitting the virus to others. Therefore, it is in the best interest of public health that any IMPs developed for SARS-CoV-2 general screening tests meet these criteria.
The second type is specific testing and will be geared toward those who are infected and are symptomatic. The pandemic has been ongoing for 20 months, and most people are now familiar with the flu-like symptoms of COVID-19. Those who experience these symptoms are more likely to seek a SARS-CoV-2 test to determine whether they have been infected. In Canada, a qRT-PCR test is performed to confirm the presence of the virus and determine the viral load. A nasopharyngeal sample is taken and sent to a government laboratory, with results available in one to three days. This is significantly longer than the Abbott Panbio™ Rapid Test and can be improved with the use of IMPs. Many microfluidic platforms that perform quantitative NAAT on viral pathogens report a turnaround time of within an hour, as seen in the ZIKV device and the SIMPLE chip
[19][20]. Shortening this turnaround time will curb the spread of the disease. Moreover, a quantitative test is preferred in this type as the viral load, which correlates to the transmissibility and severity of the virus, can assist healthcare providers in determining suitable treatment plans for patients
[21][22]. Thus, IMPs developed for specific SARS-CoV-2 testing should meet these criteria, potentially through the use of droplet microfluidics for quantification.
Serological tests, which test for anti-SARS-CoV-2 IgG and IgM antibodies, are available to test whether a person was previously infected or had gained antibodies through COVID-19 vaccinations
[23]. However, with SARS-CoV-2 and its variants fueling serious waves of new infections globally, more focus should be put into tests that determine whether someone has the virus at the time of testing, such as the ones mentioned before.
3. Conclusions
The inability of conventional diagnostic testing tools to meet the unmet demand for safely, cheaply, and reliably mass-producing accurate diagnostic tests for these devices can be seen as a limitation to controlling and monitoring the spread of highly infectious viral infections, such as SARS-CoV-2. New technologies, such as complete or partial microfluidic integration, can be used to overcome several of the limitations that are related to conventional diagnostic tools, such as the need for access to expensive laboratory equipment for sample processing. Integrated microfluidic-based diagnostic platforms have the ability to screen for and analyze patient samples in a more realistic setting without sacrificing accuracy or robustness. For example, the use of partial microfluidic integration has already shown effectiveness in diagnosing ailments, such as cancer and infectious diseases, that are not transmissible through respiratory pathways, such as the Zika virus
[21]. Additionally, near or fully integrated microfluidic platforms showed that they can effectively test for viral DNA/RNA under a wide range of conditions using a self-contained and versatile SIMPLE chip for both qualitative and quantitative analysis. Furthermore, in each case of microfluidic integration, the capacity for on-site diagnostic potential has increased. With microfluidic integration, any of the seven pillars of microfluidic integration effectively shortens the gap between unviable on-site diagnostic tests and fully realized on-site diagnostic tests. Recent advances in this continuously expanding field show strong promise in the reduction of the cost per device, the increased accuracy and modularity, the robustness, and most importantly, the speed of the sample analysis.
Nevertheless, there remain many key obstacles in facilitating the transition of our currently available diagnostic tests to mass production. The WHO’s ASSURED criteria, which defines the minimum standards for developing on-site medical diagnostic tests, can be easily achieved through microfluidic integration. For example, the global cost of producing such on-site diagnostic devices can be greatly reduced through downsizing to the microscale and the development of self-contained devices. This would be an important feature to address with microfluidic integration before the benefits outweigh the costs to mass-produce these on-site diagnostic tests to meet the current global demand. Though global costs can be reduced as more microfluidic mediums are being discovered, device sensitivity can still be limited by the diagnostic technology that is currently available to detect certain pathogens. For example, despite the spike protein of SARS-CoV-2 being well documented, there remains room for improvement in the specificity and efficiency of indicators and markers to facilitate further diagnostic improvements. Microfluidic integration can maintain the level of accuracy shown in their non-microfluidic diagnostic counterparts but will require greater feats of innovation before significant improvements to the accuracy of on-site detection methods can be achieved. Maintaining, or improving, the accuracy of IMPs is just one example of the “seven pillars” for assessing effective IMPs we have developed. On-site diagnostics of infectious pathogens, such as SARS-CoV-2, should look to a combination of the ASSURED standards and our “seven pillars” criteria to productively work toward an effective on-site diagnostic tool.
Efficient multiplexing and parallel testing so that each test can detect more than one specific pathogen is a characteristic of current on-site IMPs that has yet to be achieved in a cost-effective manner. A significant trend in developing integrated microfluidic diagnostic devices is the integration of multimode biosensor technologies to optimize data analysis and collection. Integrated microfluidic devices, combined with multimode sensing and improved algorithmic analysis, can create systems where real-time digital processing can be achieved while analyzing samples for multiple targets. In addition, there were recent improvements in droplet microfluidic technology that make use of these multimode biosensors to allow for multiplexing and single-cell analyses. Therefore, we envision that integrated microfluidic devices can achieve improved diagnostic capabilities with greater accuracy and improved detection limits with multimode sensing and improved real-time analysis software.