2. Diversity of MscS Mechanosensitive Channel Superfamily and the Impact of the MscCG Channel Gating on L-Glutamate Secretion
The existence of MscS-type mechanosensitive channels in the cytoplasmic membranes of both prokaryotic organisms, E. coli and C. glutamicum, indicates that these channels have developed through evolution to cope with changes of mechanical environments; however, its functions as an osmoregulator are significantly different [50][26]. E. coli MscS has a large open channel pore (~16 Å), and thus its conductance reaches 1 nS, whereas C. glutamicum MscCG has a significantly smaller conductance of approximately 0.3 nS [24,27,51][27][24][28]. As an osmoregulator, E. coli MscS has strong inactivation and adaptation mechanisms to mechanical stimuli that are needed to avoid the over-efflux of cytoplasmic molecules upon osmotic downshock [52,53][29][30]. However, C. glutamicum MscCG does not have these features and tends to be open as a metabolic valve rather than an osmotic safety valve [54][31]. Recent studies of the MscS channel superfamily revealed that MscS-like channels are present among cell-walled organisms, bacteria, archaea, fungi, algae, and plants, and the physiological functions of these MscS-like channels are not simply those of osmotic safety valves upon hypoosmotic shock [19]. In eukaryotes, algal and plant MscS-like channels (MSCs, MSLs) are much more complicated structures than E. coli MscS and are found in the organellar membranes of chloroplasts [32][33][34] [55,56,57] and mitochondria [58,59,60] [35][36][37] for mechanosensing. Fungal MscS-like channels (Msy1 and Msy2) are localized in the endoplasmic reticulum membranes and are involved in osmotic Ca2+ signalling upon hypoosmotic shock [61,62][38][39].
Although the physiological functions of corynebacterial MscCG-type mechanosensitive channels are still controversial, L-glutamate secretion is certainly caused by the conformational changes of the MscCG and MscCG2 channels. C. glutamicum MscCG and MscCG2 are characterized to have the N-terminal pore domain (1–286 aa), corresponding to the entire E. coli MscS that has three transmembrane helices (TM1, TM2, and TM3) and a cytoplasmic cage domain (Figure 2A). Recently, Reddy et al. refined the 3D structures of the full-length E. coli MscS embedded in lipid bilayers by cryo-electron microscopy with nanodiscs [63] [40] (Figure 2B). MscCG and MscCG2 show the highest similarity in the pore-forming helix TM3 and adjacent regions. The unique feature of the mechanosensitive channel MscCG is the C-terminal domain (287–533 aa), including a cytoplasmic loop, the fourth transmembrane helix, TM4, and a periplasmic loop. In contrast, MscCG2 does not have these features (Figure 2C). This structure is highly conserved only in corynebacteria. Several gain- and loss-of-function mutations on MscCG were identified by the glutamate productivity assay and bacterial patch-clamp technique (Figure 2C). Originally, Nakamura et al. identified W15CSLW, A100T, A111T, A111V in the N-terminal pore domain and V419::IS1207, P424L in the periplasmic loop during the screening of L-glutamate overproducing strains. These mutations were reported to cause a spontaneous L-glutamate secretion in C. glutamicum [18]. Afterwards, Nakayama et al. reported using E. coli patch-clamp that the spontaneous L-glutamate secretion was caused by the gain-of-function mutation on mechanosensitivity of MscCG channels [64][41]. These findings proved that MscCG mechanosensitivity can be evaluated by L-glutamate productivity in C. glutamicum. Using these assays, the functional domain of MscCG has been thoroughly investigated. Yamashita et al. reported, using L-glutamate productivity assay, that the N-terminal pore domain (1–286 aa) is essential to export L-glutamate, and suggested, using homology modelling, that MscCG has an extra small loop structure (221–232 aa) and its deletion resulted in the loss of channel functionality [65][42]. Becker et al. reported additional point mutations in the N-terminal pore domain and the impact of the C-terminal domain on the channel function [66,67][43][44]. A106V was the gain-of-function mutation to cause the spontaneous L-glutamate secretion, and interestingly, Q112L and V115S double point mutation caused a loss-of-function mutation, such that C. glutamicum cannot export L-glutamate, even with penicillin treatment. In contrast, the deletion of the periplasmic loop (423–533 aa) in the C-terminal domain caused spontaneous L-glutamate secretion, but not the further deletion of the periplasmic loop and the fourth transmembrane helix TM4, indicating that the fourth transmembrane helix TM4 is involved in the mechanosensitivity of MscCG channels. Moreover, Krumbach et al. further identified the gain-of-function mutations V422K, V422D, E423P, and E423S using CRSPER/Cas12a genome-editing technology [68][45]. Based on the position of these mutations, the interaction with other components of the cell wall was suggested to be crucial for MscCG mechanosensitivity.
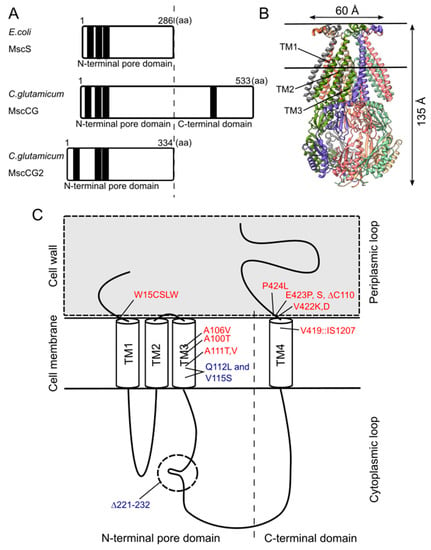
Figure 2. Structural features of the mechanosensitive channel MscCG. (A). Secondary structure comparison among E. coli MscS, C. glutamicum MscCG, and MscCG2. Black bars show predicted transmembrane (TM) helices by TOPCONS program (https://topcons.net/). (B). The cryoEM 3D structure of E. coli MscS embedded in nanodiscs with POPC:POPG = 1:4 (structure was cited from the Protein data bank 6PWP). (C). The domain structure of the MscCG channel and the gain (red)-and loss(blue)-of-function mutations.