Microalgal oils have recently been used as alternatives to fish and vegetable oils with low nutritional values [
153]. Microalgal oils contain highly utilizable and nontoxic fatty acids, such as polyunsaturated fatty acids (PUFA), arachidonic acid (ARA), c-linolenic acid (GLA), eicosapentaenoic acid, and docosahexaenoic acid (DHA) [
154]. Long-chain polyunsaturated fatty acids such as eicosapentaenoic acid (ω-3 C20:5) and DHA (ω-3 C22:6) obtained from microalgae are essential for humans due to their beneficial effects on health, including neurodevelopment and the prevention of chronic diseases [
155].
4.2. Extraction of Functional Components from Natural Resources
In addition to lipids, liquefied DME has been used to extract bioactive compounds from various sources, including spices, green tea, algae, fruits, vegetables, grains, natural plants, and fish (
Table 4). In 2003, Catchpole et al. used liquefied DME to extract specific pungent compounds from ginger, black pepper, and chili powder [
179]. Despite the significant extraction of water, liquefied DME showed similar efficacy as scCO
2 in isolating pungent compounds from spices. Complete extraction was achieved with minimal solvent consumption. At temperatures of 35, 40, 50, and 60 °C, liquefied DME showed nearly equivalent extraction rates. Subsequently, subcritical propane was suggested as a cost-effective alternative to CO
2 because of its lower operating pressure and reduced energy consumption during spice extraction, similar to liquefied DME. However, subcritical propane is the least effective at dissolving pungent components and is unsuitable for carotenoid extraction [
179,
180].
Liquefied DME has been previously used to decaffeinate green tea [
181]. The main functional components of green tea are caffeine and catechins [
182]. Excessive caffeine consumption can lead to health problems, such as dizziness, increased heart rate, tremors, and insomnia, owing to overstimulation of the central nervous system [
183]. Liquefied DME enables catechin extraction while completely removing caffeine. Ciulla et al. also demonstrated higher extraction rates of caffeine from coffee beans and powder using liquefied DME rather than using scCO
2-based extraction methods [
184].
Natural carotenoids exhibit several beneficial effects, including antioxidant, anti-inflammatory, antiproliferative, and antiapoptotic properties [
185]. As antioxidants, carotenoids detoxify intracellular free radicals, thereby reducing the incidence of oxidative damage and associated diseases [
186]. Carotenoids, which are widely distributed in nature, are biosynthesized by various organisms, including photosynthetic organisms such as algae, plants, fungi, and bacteria [
186].
Using an enzyme-assisted DME and ethanol co-solvent extraction method, Billakanti et al. were able to extract almost all lipids, including polyunsaturated fatty acids and fucoxanthin, from the wet, brown seaweed
Undaria pinnatifida [
187].
Undaria pinnatifida contains a mixture of sulfated and branched chain polysaccharides that are tightly bound to the cell wall [
188]. Therefore, extracting bioactive compounds from brown seaweed biomass is difficult because the cell wall is a major obstacle [
189].
Microalgae have attracted widespread attention as natural sources of carotenoids because they grow faster than other higher plants. The Liquefied DME extraction method successfully extracted 7.70 mg/g of astaxanthin, a carotenoid, and 30.0% of its dry weight of lipids from microalgae (
Haematococcus pluvialis) [
168]. The extraction rate of astaxanthin was 1.82% lower than that achieved through acetone extraction using drying and cell disruption. Liquefied DME extraction removed 92% of the water from the microalgae and increased the carbon and hydrogen contents. Babadi et al. reported the extraction of total carotenoids (4.14 mg/g algal dry weight) and total chlorophyll (8.45 mg/g algal dry weight) from the microalgae
Chlorococcum humicola using liquefied DME [
191].
5. Theoretical Study of Liquefied DME
The use of Hansen solubility parameters (HSP) to evaluate the solubility of various analytes of natural origin has increased [
209]. HSP is used to quantify molecular interactions and solubility [
210,
211].
HSP is based on three interaction forces: dispersion, dipole, and hydrogen bonding forces. The dispersion force (δd) indicates random interactions between molecules and represents the non-polar nature of the molecules. The dipole force (δp) indicates polar interactions between molecules and represents the polar nature of the molecule. The hydrogen bonding force (δh) represents hydrogen bonding interactions between molecules and their hydrogen bonding abilities [
212]. These interaction forces can be summed to obtain the HSP. The solubility of a substance in a solvent is higher when its HSP is similar to that of the solvent.
HSPs are typically estimated using experimental data or molecular modeling techniques [
213,
214]. The HSP distance between two substances is expressed by the following equation [
215]:
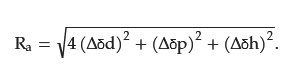
The difference in the HSP R
a [MPa
1/2] can be obtained by taking the sum of the squares of the differences between the three parameters and determining their square roots [
213]. The smaller the difference, the more similar the interactions between the substances and the higher the solubility and compatibility.
Based on the experimental data on solute–solvent interactions, plotting the solubility parameters of good and poor solvents for a solute in a three-dimensional diagram produces a Hansen solubility sphere, with regions of good solvents clustered together [
209]. The spherical region indicates the extent to which the substance interacts with the solvent. The radius of the sphere represents the interaction radius R
0 [MPa
1/2]. The ratio of R
a to R
0 is the relative energy difference (RED), which can be calculated using Equation (2). Here, RED ≤ 1 indicates a good solvent and RED > 1 indicates a poor solvent. RED can be used as an indicator of solubility [
209].
6. Bioactive Extraction to Biomedical Advances
Tuna are one of the most important marine fish species worldwide [
240]. Tuna giblets are rich in bioactive compounds such as unsaturated fatty acids, vitamins, and proteins. These compounds have antioxidant properties and can be converted into value-added products [
241]. However, the internal organs, particularly the livers, of tuna are difficult to process and are often discarded [
242].
Fang et al. reported that liquefied DME can be used to extract lipids and vitamins from tuna liver [
202]. Compared to the conventional scCO
2 method, liquefied DME extraction can prevent lipid oxidation and effectively reduce damage to omega-3 polyunsaturated fatty acids (n-3 PUFAs) and vitamins, thereby obtaining high-quality liver oil with excellent yield. The pressure used in liquefied DME extraction is much lower (0.8 MPa) than that used in scCO
2 extraction (35 MPa), and no freeze-drying pretreatment is required.
Lipids, water, and vitamins can be extracted from tuna liver using liquefied DME to precipitate high-quality proteins. Currently, pH shifts, including alkaline or acidic extraction, isoelectric precipitation, centrifugation, and lyophilization, are the best processing methods for obtaining proteins from tuna liver [
243]. However, this method is complex and time-consuming, and the lyophilization process is time- and energy-intensive [
244].
Kanda et al. crystallized glycine from an aqueous solution using liquefied DME as an antisolvent [
249]. Liquefied DME can be operated at 20–25 °C, potentially reducing the energy consumption of drying or crystallization with ethanol. Kanda et al. also prepared liposomes by dissolving soy lecithin and cholesterol in liquefied DME and infusing them into warm water [
250]. Transmission electron microscopy, dynamic light scattering for particle size distribution measurements, and zeta potential measurements revealed that the resulting liposomes ranged in size from approximately 60 to 300 nm, with a zeta potential of approximately −57 mV. This indicates that the liquefied DME injection method successfully produces liposomes similar to those produced using conventional diethyl ether at temperatures above 45 °C. The liquefied DME method does not require the residue of conventional diethyl ether in the final product of liposomes or the high-temperature and high-pressure conditions of scCO
2.
Organ transplantation is a treatment option for patients with severe organ failure. During organ transplantation, cells derived from the patient are grown on a three-dimensional scaffold to create an organ that will not be rejected. When porcine tissue is decellularized to create a scaffold, the porcine aorta is similar in structure to the human aorta, making it suitable for transplantation into humans [
251]. The decellularization of tissues from different species involves three steps: extraction of lipids using sodium dodecyl sulfate (SDS), DNA fragmentation using DNase, and the removal of DNA fragments via washing with water and ethanol [
252]. However, long processing times, inflammation caused by SDS at the contact site, and difficulty in completely removing the toxic surfactant from the tissue may cause certain problems. Liquefied DME was used to extract lipids, DNA, and cell nuclei from ostrich carotid tissue and porcine aorta [
252,
253,
254,
255]. Demonstrating that ostrich carotid tissue can be used as an alternative to porcine scaffolds, researchers can decellularize the porcine aorta after lipid extraction using DME, followed by DNase treatment and washing for at least five days. Furthermore, the introduction of liquefied DME into conventional decellularization eliminates the need for surfactants.