Lipid nanoparticles (LNPs) are spherical vesicles composed of ionizable lipids that are neutral at physiological pH. Despite their benefits, unmodified LNP drug delivery systems have substantial drawbacks, including a lack of targeted selectivity, a short blood circulation period, and in vivo instability. lipid–polymer hybrid nanoparticles (LPHNPs) are the next generation of nanoparticles, having the combined benefits of polymeric nanoparticles and liposomes. LPHNPs are being prepared from both natural and synthetic polymers with various techniques, including one- or two-step methods, emulsification solvent evaporation (ESE) method, and the nanoprecipitation method. Varieties of LPHNPs, including monolithic hybrid nanoparticles, core–shell nanoparticles, hollow core–shell nanoparticles, biomimetic lipid–polymer hybrid nanoparticles, and polymer-caged liposomes, have been investigated for various drug delivery applications.
1. Introduction
Nanoparticles (NPs) made of either polymers or lipids are widely used for the delivery of therapeutic agents. Polymeric nanocarriers are chiefly categorized as polymeric micelles, polymer–drug conjugates, and polymeric NPs, while lipid-based nanocarriers are distinguished as liposomes, solid lipid nanoparticles (SLNs), and nanostructured lipid vectors
[1][2][1,2]. Polymeric nanoparticles (PNPs) are currently gaining popularity in biomedical research, possibly due to their numerous attractive properties, including biocompatibility, biodegradability, the ease of processing, and sustained release patterns of incorporated drugs
[3]. PNPs are easy to prepare with simple and reproducible synthesis processes, with ample flexibility for chemical modifications
[4]. Polymeric NPs usually have low polydispersity and high stability. However, there are certain limitations associated with using polymers as nanocarriers for drug delivery, for instance, the use of harmful organic solvents in the preparation, the expensive synthesis, the scale-up process, the toxicity of degradation products, and the usually low drug loading capacity
[5][6][5,6]. Moreover, the hydrophobic surface of PNPs is recognized as a foreign material that provokes an immune response and prompts rapid elimination from the body, which limits their use. Furthermore, the limited cellular membrane permeability of PNPs leads to poor transfection efficiency
[7][8][7,8].
Lipid nanoparticles, similar to PNPs, have piqued the interest of researchers in recent decades and have had remarkable clinical success in delivering drugs, nucleic acids, and vaccines. Lipid nanoparticles offer numerous attractive benefits, including low production costs with an ease of preparation, an improved drug-entrapping efficiency, great biocompatibility, feasibility of scale-up, and targeted delivery. However, they have abridged stability, fast drug release, and excessive polydispersity, which are the major limitations for their widespread use
[9][10][11][9,10,11].
To overcome these limitations of PNPs and lipid nanoparticles, a new system called lipid–polymer hybrid nanoparticles (LPHNPs) has been developed owing to the biomimetic and biocompatible advantages of this strategy. LPHNPs take advantage of the benefits of both polymeric and lipid-based systems
[12]. LPHNPs are next-generation core–shell nanosystems conceptually formed from a polymer core wrapped by a lipid layer. Despite their widespread attention, they are not yet widely used or pervasive
[13]. LPHNPs have three structural features, including (
Figure 1):
Figure 1.
General structure of LPHNP composed of a drug-encapsulating polymer core with an outer lipid shell and an outer lipid–PEG layer.
- (i)
-
A drug encapsulating polymer core;
-
- (ii)
-
A lipid layer surrounding the polymer core;
-
- (iii)
-
An outer lipid–PEG layer.
-
The lipid layer acts as a molecular barrier, reducing drug loss during LPHNP formulation and further protecting the core from degradation by blocking water diffusion into the inner core. The outer lipid–PEG layer prolongs the blood circulation of LPHNPs by suppressing the immune response
[14].
2. LPHNPs Composition and Its Influence
LPHNPs composed of natural, semi-synthetic, and synthetic polymers (i.e., chitosan, PCL, polycaprolactone; PEG, polyethylene glycol; PLA, polylactic acid; PLGA, polylactic-co-glycolic acid; PbAE, poly(β-amino ester); etc.) serve as solid core NPs coated with lipids (i.e., palmitic, myristic, and stearic acids, etc.). The simplest design of LPHNPs has a drug-entrapped hydrophilic or hydrophobic polymer core surrounded by a lipid layer. Lipid shells can be easily modified to obtain the desired surface properties which promotes, for instance, efficient uptake, controlled drug release, and favorable biodistribution. The lipid composition can be easily modified and exploited to expedite the covalent or noncovalent attachment of drugs, ligands, transferrin, antibodies, folic acid, aptamers, and bioactive molecules, including nucleic acids or proteins. Lipid shells have the additional advantage of charged or zwitterionic lipids, which may promote self-assembly of the lipid layers with opposite charges on the polymeric core through electrostatic interactions. Likewise, the uses of the lipophilic core endorse its interaction with the hydrophobic tail domain of the lipids constituting the lipid layer
[15][26].
Table 1 presents the polymers and lipids used in the preparation of LPHNPs.
Table 1.
Commonly used polymers and lipids for the preparation of LPHNPs with their structures.
Polymer Used |
Lipid Used |
Reference |
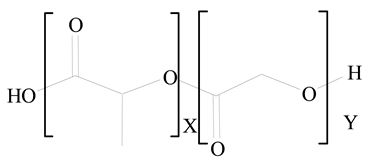 Polylactic-co-glycolic acid (PLGA) |
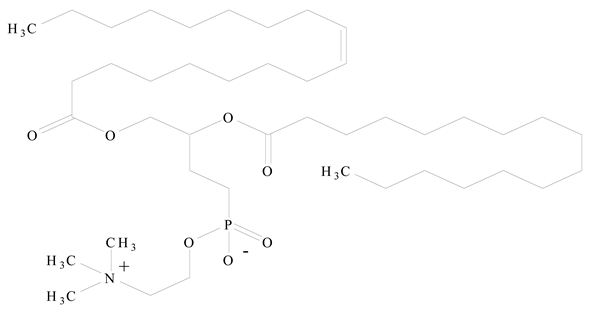 Phosphatidyl choline (lecithin) |
[16][27] |
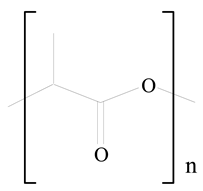 Polylactic acid (PLA) |
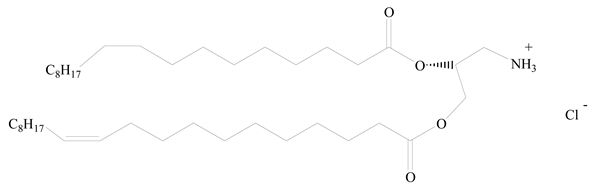 1,2-Dioleoyl-3-trimethylammonium-propane (DOTAP) |
[17][28] |
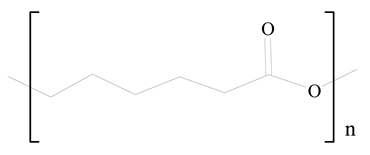 Polycaprolactone (PCL) |
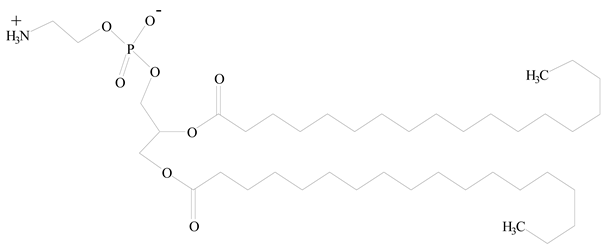 1,2-Distearoyl-sn-glycero-3-phosphorylethanolamine (DSPE) |
[18][29] |
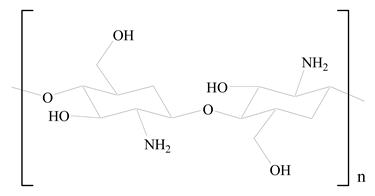 D-Glucosamine and N-acetyl-D-glucosamine (chitosan) |
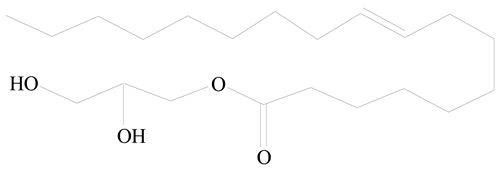 Glyceryl monooleate (GMO) |
[19][30] |
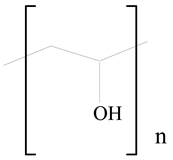 Poly(vinyl alcohol) (PVA) |
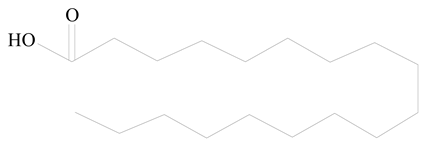 Stearic acid |
[20][31] |
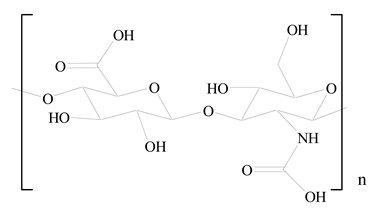 Hyaluronic acid (HA) |
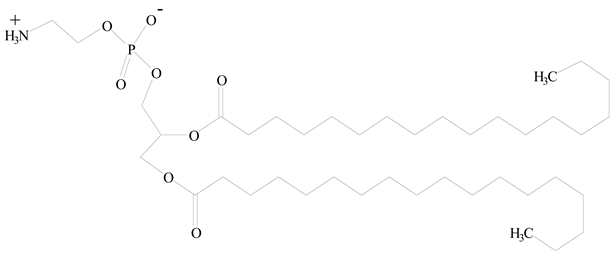 1,2-Distearoyl-sn-glycero-3-phosphorylethanolamine (DSPE) |
[21][32] |
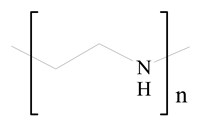 Polyethylenimine (PEI) |
 1,2-Dioleoyl-sn-glycero-3-phosphoethanolamine (DOPE) |
[22][33] |
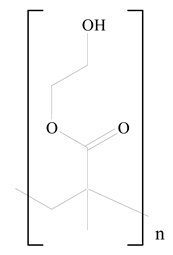 Poly(2-hydroxyethyl methacrylate) (PHEMA) |
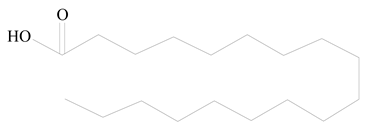 Stearic acid |
[23][34] |
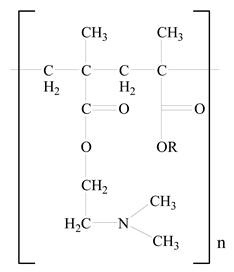 Eudragit |
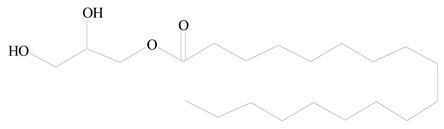 Glycerol monostearate |
[24][35] |
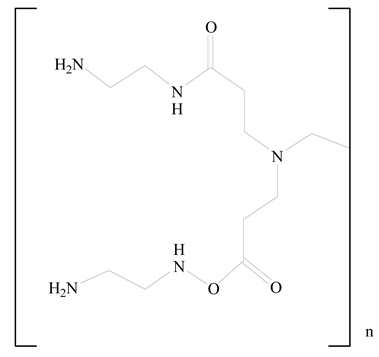 Polyamidoamine |
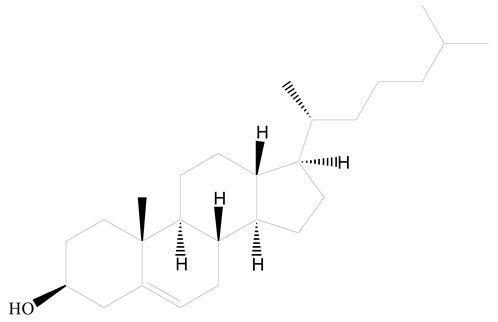 (3β)-Cholest-5-en-3-ol (cholesterol) |
[25][36] |
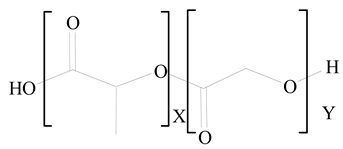 Polylactic-co-glycolic acid (PLGA) |
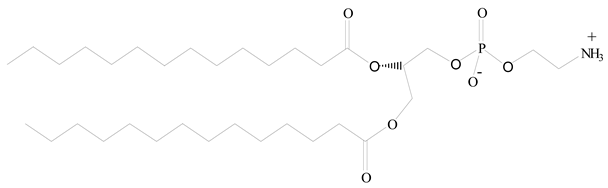 1,2-Dimyristoyl-sn-glycero-3-phosphoethanolaminediethylene (DMPE) |
[26][37] |
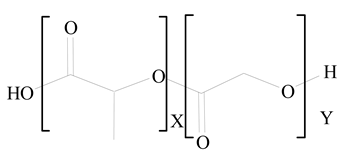 Polylactic-co-glycolic acid (PLGA) |
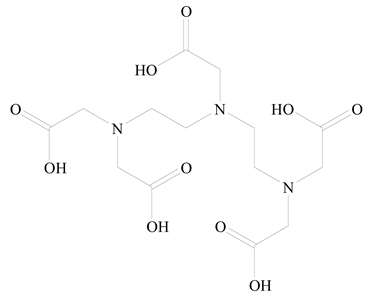 Diethylenetriaminepentaacetate (DTPA) |
[26][37] |
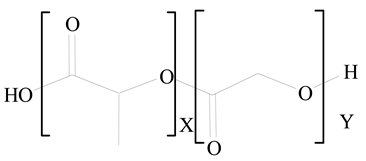 Polylactic-co-glycolic acid (PLGA) |
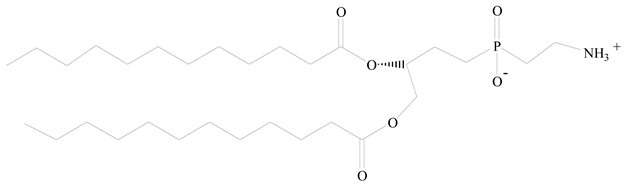 1,2-Dilauroyl-sn-glycero-3-phosphocholine structure (DLPC) |
[27][25] |
Polymer aggregation and intrinsic viscosity have a direct influence on the particle size. Higher polymer concentrations yield larger particles, while polymers with a higher intrinsic viscosity produce smaller particles
[28][38]. The surface zeta potential of LPHNPs can be altered by changing the end functional groups on shells (usually PEG). Because surface charges prevent particles from colliding, higher absolute zeta potential values result in more stable NPs in vitro. Dave et al.
[29][39] reported that on increasing the concentration of soy lecithin from 20 mg to 30 mg, the zeta potential of the prepared LPHNPs also increased from 23.4 ± 1.5 mV to 41.5 ± 3.4 mV. Furthermore, the interaction of charge inducer (i.e., stearyl amine, SA) with the lipid surface also alters the zeta potential of formulations. According to immunocompatibility tests, the complement activation is the lowest in hybrid NPs with methoxyl surface groups and the highest in NPs with amine surface groups
[30][40]. An adequate surface charge must be chosen to match the hybrid NPs in vitro stability and in vivo immunocompatibility. The lipid-to-polymer ratio (L/P) had a significant impact on the colloidal stability of hybrid particles. The lipid layer may operate as an electrostatic stabilizer when the lipid-to-polymer L/P ratio is high and the cationic lipid concentration is high. At low L/P and cationic lipid fractions, the partial lipid coating of the polymer core was inadequate to colloidally stabilize the LPHNPs. For example, when the anionic surface of one hybrid molecule core was exposed to the cationic 1,2-dipalmitoyl-3-trimethylammonium-propane, LPHNPs aggregation occurred. Surprisingly, when 1,2-dipalmitoyl-sn-glycero-3-phosphocholine (DPPC) was employed alone, the hybrid NPs were less prone to agglomeration. This is because the DPPC’s zwitterionic structure lowers the possibility of electrostatic interactions
[31][41].
Poly(lactic acid) (PLA) is a biopolymer that has gained more attention because of its both biodegradable and biocompatible nature. Because of its high safety profile, the US Food and Drug Administration (US FDA) has approved it for a variety of purposes. For almost two decades, LP-anchored PLA NPs have been employed to deliver medicines, peptides, and vaccinations
[32][42]. The greater mechanical stability of LPHNPs during storage and in serum is attributed to the polymeric core, which behaves as cytoskeleton and to a PEG-coated lipid layer, which improves blood circulation time in vivo by suppressing the immune system
[33][43]. However, the low cell interaction leads to a poor drug delivery efficiency, which is the major limitation. The problem can be resolved by coating or attaching a site-specific target ligand with an outer lipid layer of NPs to mimic the binding with cell surfaces and deliver the payload. The biomimetic LPHNPs retain both the physicochemical features of the synthetic vehicles and inherit the intrinsic functionalities of the cell membranes
[34][44].
Lipids are amphiphilic or hydrophobic molecules and are present in numerous compounds, including fatty acids, oils, steroids, and waxes. Glycerophospholipids are the most common type of biological membrane component, consisting of a glycerol molecule, a phosphate group (PO
42−), and two fatty acids. These phospholipids are broadly used for the surface engineering of PNPs. Phospholipids, including phosphatidylcholine, phosphatidylglycerol, phosphatidylinositol, phosphatidylserine, phosphatidylethanolamine, and phosphatidic acid are less stable in nature. Thus, synthetic lipids have been reported through the modification of the polar and nonpolar regions of the phospholipid molecules. Synthetic phospholipids, including PEGylated phospholipids, 1,2-diacyl-P-O-ethylphosphatidylcholine, etc., are cationic, anionic, neutral, and zwitterionic phospholipids and are often used in biomedical engineering
[35][45]. Both natural and synthetic lipids have been used for the surface anchoring of PNPs. Natural or cell-membrane-derived lipids have the advantages of natural cell surface, natural targeting ability, natural immune-evading property, long circulating half-life, and well-controlled tissue distribution. Synthetic lipids have the advantages of biomimetic surface, controlled drug release, stealth properties, extended circulation, and surface flexibility for targeting functionalization
[31][41].
The lipid-based surface functionalization of PLGA NPs is showing encouraging outcomes in the fabrication of PLGA-based nanomedicines due to their fascinating properties of biocompatibility, biodegradability, ease of treatment, and sustained release. The targeting specificity of PLGA-based nanocarriers can be enhanced by surface engineering with various lipids. This may further improve the physicochemical properties of nanocarriers as well as their NP–cell associations, including cellular membrane permeability, immune responses, and prolonged blood circulation. PLGA-based LPHNPs have been reported to be utilized for drug and gene delivery
[36][46].