1. Introduction
As aerospace and automobile industries progress, the demand for materials with superior strength and toughness increases. However, strength and toughness, two crucial properties governing the performance and failure of metal components, often show an inverse relationship. This means that improving strength can compromise fracture toughness, posing an ongoing challenge for material scientists. One effective approach to enhance metal materials is the creation of composites
[1[1][2],
2], which involves incorporating high-performance particles into the metal matrix. These metal matrix composites (MMCs) offer notable improvements in specific strength, stiffness, wear resistance, and other desired properties
[3,4,5,6][3][4][5][6]. Additionally, they possess advantageous characteristics such as high conductivity, thermal conductivity, damping, wear resistance, and multi-functionality, making them valuable for future applications in metal structural materials
[7,8,9,10,11][7][8][9][10][11].
In aerospace, electronics, and electric vehicles (EVs), the use of lightweight advanced metals, particularly metal matrix composites (MMCs), offers a strategic advantage due to their light weight, high strength, and high toughness. Particularly in the aerospace industry and spacecraft development, advanced aluminum matrix composites (AAMCs) are important base materials that enable cost-effective, multi-functional solutions while meeting fuel efficiency and sustainability goals. This innovative approach to aerospace engineering provides reliable solutions to current challenges and future design requirements. MMCs possess important properties such as high structural efficiency, excellent wear resistance, and desirable thermal and electrical properties that make them valuable in various industries such as automotive, rail, thermal management, aerospace, industrial, recreational, and infrastructure
[12]. Replacing conventional aluminum alloys with high-strength and high-toughness aluminum matrix composites (AMCs) can reduce structural weight by about 20% to 25%, while increasing energy performance by about 15% to 20%
[2].
Composite configurations serve as the basis for creating sophisticated materials that transcend the limitations of individual parts. These configurations are strategically designed arrangements of different phases, materials, and microstructures that are integrated to achieve specific mechanical, electrical, thermal, and functional properties. The importance of composite configurations lies in their profound influence on material behavior. They enable the production of high-performance materials that meet the requirements of modern engineering applications. Composite configurations can be adapted to different requirements. By intelligently designing the arrangement of the individual phases, scientists can select the desired properties while mitigating limitations. For example, incorporating reinforcing phases into MMCs can dramatically increase stiffness and strength, enabling the material to withstand demanding mechanical loads. Similarly, the incorporation of thermally conductive phases into polymer-based composites can contribute to good thermal conductivity, which is important for applications such as the electricity industry. In addition, composite configurations allow for the manipulation of mechanical performance characteristics such as toughness, fatigue resistance, and fracture behavior. By combining these methods, researchers can stop crack propagation, deflect crack paths, and improve energy dissipation mechanisms, thereby increasing fracture toughness and overall mechanical integrity. This design freedom also extends to tailoring anisotropic behavior, where material properties are varied in different directions to allow for optimal operation under specific conditions. In addition, composite configurations combine different components into a single material system, allowing the materials to perform multiple functions simultaneously. Beyond performance benefits, composite configurations contribute to sustainable design principles by reducing material consumption and weight, thus minimizing environmental impact. In essence, composite configurations represent the cutting edge of material development. Through the use of microstructures and interfaces, composites offer unprecedented capabilities, performance and stability.
2. Strengthening and Toughening of Micro-Heterogeneous Configurations of Reinforcements
A design configuration consists of microelements that interact to form mesoscopic objects that act as building blocks within the RVE
[55][13]. When we talk about heterogeneous configurations of gain elements, we refer to the relationship between micro- and nano-scale gain elements and their distribution, size, shape, and other structural parameters relative to the matrix material. The terms network, micro–nano, and micro–nano hybrid architectures are used to describe the design scale and configuration. Common to all is the uniform distribution of macro- and micro–nano-scale gains within a given engineered structure. AMMCs illustrate this concept by exhibiting improved grain boundary efficiency compared to base materials or those with crystalline particles. This improvement is attributed to the presence of a three-dimensional network of closed alumina cells at the micro-scale filled with elastoplastic aluminum. This network effectively prevents restoration mechanisms across grain boundaries and grain boundary sliding during hot working, resulting in an extremely stable UFG structure with superior thermal and strain stability
[56,57,58][14][15][16]. Such macro-heterogeneous structures hold great potential for the development of materials with desirable properties suitable for load-bearing structural applications, especially at high temperatures.
Previous studies
[59,60,61][17][18][19] have shown that the design approach for network configurations has changed from continuous reinforcement networks to quasi-discontinuous and quasi-continuous networks. The original design of the (Al
3Zr + Al
2O
3np)/2024Al continuous network composite exhibited concentrated and agglomerated regions of reinforcement along the grain boundaries of the matrix, resulting in significant stress concentration and limited balance between strength and toughness. Attempts to adjust the sintering temperature, pressure, and other process parameters did not result in a satisfactory balance between strength and toughness
[62][20]. On the other hand, configurations with quasi-continuous or continuous reinforcement networks, such as SiC/Al composites with a 3D quasi-skeleton (interpenetrating network structure), have shown remarkable improvements in thermophysical properties. The geometry of the SiC reinforcement has a significant impact on these properties, and the co-continuous structure of the SiC reinforcement and the Al matrix in 3D SiC/Al composites has been shown to be particularly advantageous
[59][17]. These configurations not only exhibit excellent homogeneity, but also show improved mechanical and elastic properties due to their unique structure and strong interfacial bonding between the matrix phase and the reinforcing phase
[60,61][18][19].
Compared to conventional composites using particles or fibers as reinforcing materials, composites with an interpenetrating phase network exhibit improved toughness and isotropic microstructures. In MMCs with a quasi-continuous 3D network structure, the interface between the continuous ceramic and metal phases is smaller, resulting in lower interfacial thermal resistance than partially reinforced MMCs with the same ceramic volume fraction
[59][17]. The strategic distribution of reinforcements within a network or quasi-continuous skeleton can simultaneously improve the strength and toughness of titanium matrix composites at room temperature. In addition, the incorporation of a discontinuous three-dimensional graphene network into copper-based composites improves their ability to accommodate geometric dislocations, resulting in improved mechanical properties such as increased elastic modulus, yield strength, tensile strength, elongation at break, and fracture toughness
[63][21]. This type of network configuration provides a uniform distribution of reinforcement throughout the matrix and ultimately improves the strength and toughness properties of the metallic composite. In addition, the optimization of structural parameters such as the size, shape, and distribution of reinforcement is expected to further improve the strength and toughness properties.
The nano-micron hybrid configuration is an effective design that combines two different sizes of reinforcements to synergistically improve both strength and toughness under load. Larger particles can withstand significant loads and accumulate strain energy, triggering recrystallization in the surrounding metal matrix
[17,64,65,66][22][23][24][25]. On the other hand, smaller nanoparticles reduce the stress concentration in the GBAZs and IAZs around the larger particles, retarding phenomena such as recrystallization and grain growth (
Figure 21)
[3,67,68,69,70][3][26][27][28][29]. The uniform distribution of reinforcing elements in two different sizes is challenging in conventional fabrication processes, especially for nanocarbon materials such as carbon nanotubes and graphene, limiting the achievement of desired toughness and plasticity in nano/micron hybrid configurations
[71,72,73,74,75][30][31][32][33][34].
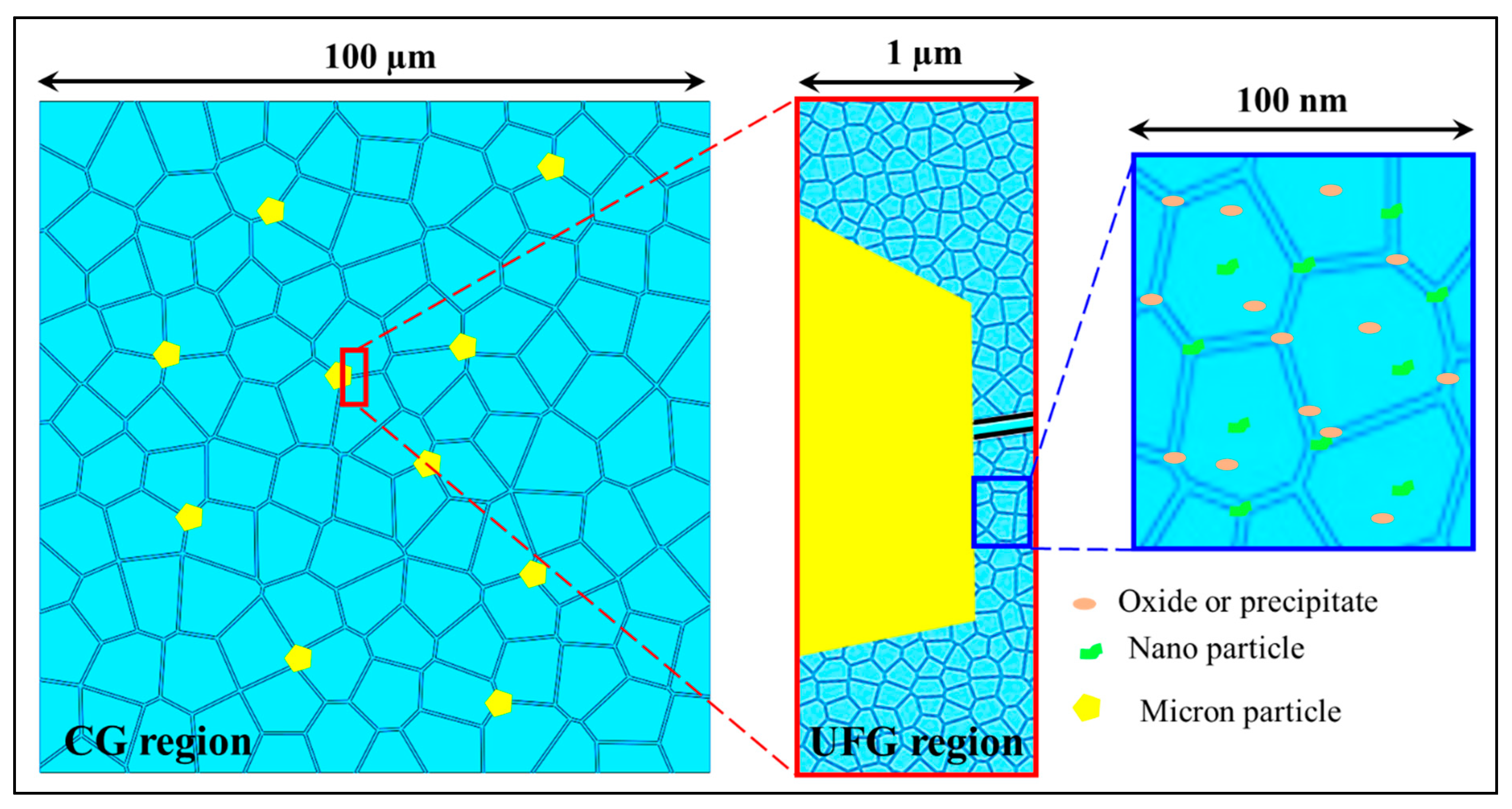
Figure 21. Schematic of the micro–nano hybrid architecture; size-dependent design and cooperative dispersion of micron particles and nanoparticles in the micro–nano hybrid configuration.
In order to identify a suitable fabrication technique, step-by-step powder metallurgy, also known as powder assembly, has emerged as one of the most effective and practical methods for achieving the essential structural parameters and harnessing the synergetic effects of reinforcements with different size scales in composites. Employing this approach, the (B
4Cp+Al
2O
3np)/Al micro–nano hybrid composite has been successfully produced, exhibiting an elongation-to-fracture of approximately 8.9%. This value surpasses the elongation-to-fracture of 4% observed in B
4C/Al composites reinforced solely by micron-scale reinforcements. The presence of sub-micron-sized Al
2O
3 nanoparticles within the aluminum grains, forming Orowan loops in the grain interiors, significantly contributes to the hardness and facilitates uniform plastic deformation
[43][35]. Furthermore, the adoption of a reinforcing design configuration leads to enhanced fatigue and strength properties in bimodal-sized Al
2O
3/Al nanocomposites, which incorporate both micro-sized and nano-sized Al
2O
3 particles. The uniform presence of nano-sized alumina within the grain interior restricts dislocation mobility, while the micro-sized alumina at the grain boundaries limits grain boundary migration
[17][22].
The incorporation of nanoparticles as reinforcements within the matrix grains surrounding micron-sized reinforcements offers a significant enhancement in both toughness and tensile strength. In specific cases, the addition of sub-micron Al
2O
3 particles in nano/micron hybrid composites, such as (Al
2O
3p+AlN-np)/Al, facilitates the dispersion of AlN nanoparticles. The presence of sub-micron reinforcements activates a portion of the matrix grains, leading to the recrystallization and reorganization of stored dislocations, resulting in simultaneous increases in strength and toughness
[58,76,77][16][36][37]. Generally, achieving the uniform dispersion of nanoparticles is a vital concern for making nanoparticle-reinforced composites as it directly impacts the mechanical, thermal, and other functional characteristics of the composite. Here is a list of some common approaches used to ensure homogeneous dispersion of the second phase within the matrix:
-
Mechanical Mixing: Utilizing mechanical methods such as ball milling, attrition milling, and high-energy mixing can help break down agglomerates and promote a more even distribution of the second-phase particles within the matrix.
-
Ultrasonic Dispersion: Ultrasonic waves can be employed to disperse and break down clusters of second-phase particles, ensuring their uniform distribution within the matrix material.
-
Powder Metallurgy (PM) Techniques: Powder metallurgy methods, such as hot isostatic pressing (HIP) and spark plasma sintering (SPS), involve consolidating powders under controlled conditions. These methods can result in a more uniform distribution of the second phase due to the homogenizing effect of powder compaction
[64][23].
-
Liquid Phase Infiltration: Introducing a liquid matrix into a preform of reinforcing particles can help achieve a uniform distribution of the second phase, especially in cases where the reinforcing phase is in a fibrous or porous form.
-
Gradient Precipitation Techniques: Controlling the cooling rate during solidification can promote the uniform precipitation of the second phase, resulting in a more homogeneous distribution.
-
Surface Treatment and Coating: Treating or coating the second-phase particles before incorporation can improve their wetting and dispersion within the matrix material.
-
Electrostatic Dispersion: Employing electrostatic forces can assist in dispersing and separating particles, enhancing their uniform distribution within the matrix.
-
Additive Manufacturing: Techniques such as 3D printing allow precise control over the placement of the second-phase material, enabling the creation of complex geometries with uniform distribution.
-
Melt Infiltration: For fiber-reinforced composites, melt infiltration involves impregnating a porous preform with molten matrix material. This can facilitate a more even distribution of the matrix around the fibers.
-
Controlled Agitation and Stirring: Utilizing controlled agitation or stirring during the mixing and consolidation processes can prevent settling and promote uniform distribution.
-
Sol-Gel Techniques: Sol-gel processes can result in the formation of a homogenous precursor material that can be used to achieve uniform distribution upon consolidation.
-
Smart powder processing: In this method, the process involves utilizing four distinct mechanical devices to induce mechanical forces, leading to the covering of fine shell (guest) particles onto the surface of larger core (host) particles, which in turn develop the advanced production of materials
[71,78][30][38].
These ideas can be based on the specific type of composite, the composition of the secondary material, and the desired end product. A combination of these methods can also be used to obtain a good view of the second phase in the matrix material. However, achieving the uniform distribution of high-modulus and high-strength nanocarbon reinforcements such as CNTs and graphene in composites remains a challenge for the development of advanced MMCs. The adverse effects, such as lateral reactions and formation of metal carbides at the interfaces, may compromise the structural integrity of CNTs or graphene due to excessive energy input during ball milling during the initial fabrication process. Therefore, minimizing the energy input is proposed as a solution
[46][39]. Moreover, the enrichment of nanocarbon reinforcements significantly affects the plastic behavior of the resulting composite. For example, significant increases in elastic modulus, yield strength, tensile strength, and elongation at break were observed in a (SiCp+CNT)/6061Al nano-micron hybrid composite fabricated by mechanical milling in which CNTs were grown in situ on the micrometer surface of SiC particles
[47,48][40][41]. The use of a predispersion process, such as high-shear predispersion, has also been shown to be effective in predispersing nanocarbon on the surface of platelet Al powder and providing enhanced protection in subsequent mechanical powder processes
[71,79][30][42]. A well-coordinated CNT dispersion, structural integrity, and interfacial adhesion in a fabricated 1.5 wt.% CNT/6061Al composite demonstrated improved tensile toughness. These results underscored the importance of structural designs that ensure the reasonable distribution of multi-phase reinforcements while considering the optimal conditions for achieving desired composite properties. The excessive volume fraction of reinforcements in the metal matrix should be avoided, as this may lead to stress concentration at the interfaces and in the GBAZ, resulting in poor mechanical behavior in terms of strength and toughness. In summary, the shape and size of reinforcements play a crucial role in achieving high strength and toughness in composites. It is worth noting that the toughness of composites has an inflection point or reversal at a certain stage.
Inspired by the micro–nano brick structure found in nacre (mother of pearl)
[21][43], efforts have been undertaken to develop a similar configuration in layered composites. This configuration involves the distribution of reinforcements with varying size scales, shapes, and distributions within the matrix. Similar to the brick-and-mortar arrangement, brick-shaped mineral platelets at the micro-scale are embedded in a matrix of nano-scale material. The utilization of flake powder metallurgy technology has played a crucial role in achieving precise control over the configuration parameters in layered composites
[73,80][32][44]. By producing platelets in two dimensions, this approach ensures better geometric compatibility, leading to a more uniform distribution of reinforcements. Furthermore, the elongated grains with an increased aspect ratio (width in the nanometer range and length in the micrometer range) enable the storage of dislocations. This facilitates the intentional placement of in situ Al
2O
3 nanoparticles, CNTs, graphene plates, and other reinforcements at the grain boundaries of the elongated grains. As a result, a high density of geometrically necessary dislocations (GNDs) is formed near the grain boundaries and interfacial zones, compensating for the loss of strain hardening caused by ultra-fine grains
[81,82][45][46].
Various techniques have been employed to enhance the mechanical entanglement and load transfer effect at interfaces in composite materials. These methods include combining powder metallurgy (PM) with conventional rolling
[3[3][31],
72], electrophoretic deposition of CNTs on metal foil
[83][47], constructing biomimetic mineral bridges, and in situ reinforcement growth
[84][48], among others. By improving the interfacial bonding and structural quality of the reinforcement, these techniques require more energy for crack propagation, resulting in a significant increase in toughness.
The geometric compatibility between two-dimensional graphene plates and flake-shaped building blocks formed in the flake PM process makes graphene an ideal reinforcement for biomimetic brick matrix structures. For example, in the GNS/Al micro–nano brick composite, the geometric compatibility of graphene plates with flake-shaped platelets prevents the annihilation of dislocations at the grain boundaries
[85,86][49][50]. This composite demonstrates an increase in tensile strength from 201 MPa to 302 MPa, while maintaining a similar level of elongation to fracture.
Recently, a new design approach for high-performance Ti
2AlC/TiAl composites with a micro–nano-laminated configuration has been explored. This design exhibits a favorable combination of compressive strength (1807 MPa) and ductility (25.5%) at room temperature
[87][51]. The composite consists of microlaminated Ti
2AlC, micro–nano-γ-TiAl/α2-Ti3Al lamellar colonies, and nano-laminated Ti
2AlC. These structural features contribute to the increased strength of the Ti
2AlC/TiAl composite through load transfer reinforcement and Orowan strengthening effects commonly observed in metal matrix composites. In addition, this design configuration improves the ductility and toughness of the Ti
2AlC/TiAl composite through several plastic deformation mechanisms
[87][51]. The configuration of a design, including the size and shape of its components such as grains and particles that provide reinforcement, plays a critical role in the ability to store dislocations and the likelihood of defects forming in the structure. The response to high strain rates is influenced by the constraints imposed by the geometry of the reinforcement
[48,73,88][32][41][52]. In addition, the interaction between intrinsic mechanisms (related to plasticity) and extrinsic mechanisms (related to shielding) to increase toughness is strongly influenced by the design configuration
[89,90,91][53][54][55]. Intrinsic mechanisms act primarily on small scales (in the nanometer or micrometer range) upstream of the crack tip and enhance the material’s inherent resistance to damage, thus increasing both crack initiation and propagation toughness. In contrast, extrinsic mechanisms produce a shielding effect at larger length scales, usually behind the crack tip, and only affect toughness during crack propagation
[92][56]. Essentially, intrinsic toughness relies on plasticity to increase a material’s resistance to damage, resulting in improved crack initiation and propagation toughness. On the other hand, extrinsic toughness reduces local stresses and strains at the crack tip and is effective only in the presence of a crack, affecting crack propagation toughness. In this context, the combination of multi-scale extrinsic toughening mechanisms
[35,83,93][47][57][58] induced by the reinforcements and the matrix can significantly contribute to increased strain hardening and the formation of exceptional strength and toughness.
In configurations featuring micro-scale heterogeneity of reinforcements, incorporating nanosized reinforcements alongside sub-micron and micron reinforcements leads to improvements in strength and toughness across various size scales, ultimately enhancing the balance between stability and toughness. The utilization of nanoscale reinforcements in future advanced composites is considered crucial, as indicated by numerous studies
[94,95][59][60]. For instance, a boron carbide (sn-B
4C)/Al composite with spatial arrays of sn-B
4C demonstrated a significant increase of 26% in tensile strength and 30% in toughness. This enhancement can be attributed to the presence of fiber-like nanoparticle-rich (NPR) zones, acting as “hard” fiber-like units, effectively sustaining tensile loading and enhancing the strengthening efficiency of sn-B
4C. Furthermore, the arrangement of NPR zones, surrounded by nanoparticle-free (NPF) zones as the softer phase, contributes to improved strength with minimal loss in ductility
[94,95,96][59][60][61].
The reinforced design configuration, particularly the fiber-like distribution of reinforcements, introduces additional microstructural strengthening through dislocation strengthening and the activation of Orowan mechanisms. The presence of spherical nanoparticles within the NPR zones immobilizes more dislocations, leading to a significant disparity in hardness values between the NPR and NPF zones. This disparity enables stress transfer from the “soft” NPF zones to the “hard” NPR zones, resulting in the extraction of some fiber-like NPR zones at the fracture surface during tensile loading. However, challenges persist in achieving uniform distribution and limited work hardening in ultra-fine grains. Controlling the spatial distribution of reinforcing particles at the micro- and meso-scale to engineer distinctive microstructural configurations has emerged as an innovative approach to overcome existing trade-offs in material properties
[36,97,98][62][63][64].
Considering the toughness of micro-heterogeneous reinforcement configurations, it is clear that refining the interaction between the matrix and the reinforcement phase can lead to remarkable improvements in the toughness of CNT- or graphene-reinforced MMCs. Yizhang Liu et al.
[99][65] discussed the development stage of ceramic matrix composites reinforced with nanocarbon materials. The study investigated the influence of interfacial bonding conditions on the fundamental properties of these composites and explored strategies to improve this interfacial bonding. Afifah Md Ali et al.
[100][66] addressed three key topics on the challenges associated with the use of graphene as a reinforcing material. These topics included the mechanisms by which graphene increases the strength of metal matrices and the constraints that limit the extent of property enhancement. Surface modification techniques applied to the reinforcing phase can optimize interfacial adhesion. Functionalization of the surfaces of CNTs or graphene with suitable chemical groups can improve compatibility with the matrix, ensuring robust load transfer and preventing premature detachment. Rama Dubey et al.
[101][67] investigated how modifying the surfaces of CNTs can optimize their interaction with other materials. Introducing specific chemical groups into CNT surfaces increased their compatibility with surrounding matrices, resulting in robust load transfer and prevention of premature detachment. In addition, the development of hybrid structures in which CNTs or graphene are coupled with secondary reinforcements such as nanoparticles or fibers can result in multiple toughening mechanisms. This synergistic combination of reinforcing elements leads to mechanisms such as crack deflection by secondary phases, crack fixation by nanoparticles, and stress redistribution by fiber bridging. The resulting interactions mitigate crack propagation and contribute to the overall improvement in toughness. Jyoti et al.
[102][68] investigated the field of hybrid structures where graphene or carbon nanotubes were combined with additional reinforcements such as nanoparticles or fibers. This integration resulted in various toughening mechanisms, including the deflection of cracks by secondary phases, immobilization of cracks by nanoparticles, and redistribution of stresses by fiber bridging. The combined effect of these mechanisms impedes crack propagation and increases the overall toughness of composites.
It is important to note that both macro- and micro-scale configurations of reinforcing elements significantly affect the balance between toughness and strength in metal composites. However, achieving a satisfactory spatial distribution of reinforcements requires careful consideration. For example, achieving uniform intragranular dispersion of CNTs in an aluminum matrix requires specific conditions related to time, energy, external loading, and thermal activation
[103,104][69][70]. In addition, challenges remain in overcoming the trade-off between strength and toughness, highlighting the need for further extensive research in the area of strengthening and toughening mechanisms. In summary, the relative decrease in toughness observed in CNT- or graphene-reinforced MMCs requires a dual strategy involving both macro- and micro-heterogeneous configurations of reinforcements. Strategic design choices, such as gradient distributions, sacrificial phases, surface functionalization, and hybrid structures, can leverage toughness enhancement mechanisms to significantly improve the overall performance of these advanced composites.