Values of “x” vary depending on annealing temperature and oxygen partial pressure during synthesis. As a result, oxygen vacancies form in the oxygen sub-lattice and n-type semiconducting is induced. To be neutral, some V
5+ ions will be reduced to V
4+ ions. Electrical conductivity in V
2O
5−x is due to jumping (hopping) of the electrons from V
4+ ions to the neighboring V
5+ ions
[26].
Compared with bulk V
2O
5, nanostructured V
2O
5 materials have unique electrical and chemical properties and due to their ultrafine sizes, they offer a high surface area which is extremely beneficial for sensing studies
[27][28][29][30].
Nowadays gas sensors have become widely used in different areas for detection of toxic, hazardous, explosive and greenhouse gases and vapors
[31][32]. There are many types of gas sensors. However, resistive-based gas sensors using metal oxides are the most widely used gas sensors due to their unique features such as low price, high sensitivity, high stability, fast dynamics and simple fabrication and operation
[33][34]. In a typical gas sensor, the front side of substrate is equipped with conductive electrodes such as with Pt and its back side is equipped with a heater
[35].
Pristine V
2O
5 gas sensors with different morphologies have been reported in the literature. The gas sensing characteristics of V
2O
5 hierarchical architectures, especially for hollow spheres are rarely reported in the literature. In this regard, V
2O
5 hollow spheres (500–550 nm in diameter and a shell thickness of 55 nm) were synthesized through a solvothermal route. The hollow spheres were comprised of nanoplates with thicknesses of 50–80 nm and lengths of 70–120 nm. Moreover, for comparison solid nanostructured spheres were fabricated. The maximum responses (R
a/R
g) to trimethylamine (TEA) at 370 °C were 9.7 for V
2O
5 hollow spheres and 3.08 for V
2O
5 solid spheres, respectively. In fact, the hollow sphere hierarchical architecture with a higher surface area offered more adsorption sites for TEA molecules and a higher response for hollow spheres resulted. The sensing mechanism of the sensor to TEA was related to the reduction of V
5+ ions to V
4+ ions in the presence of TEA. Furthermore, based on XPS studies there was a slight shift to lower binding energy values after exposure of the sensor to TEA gas, confirming formation of V
4+ ions. In addition, a color change from yellow to dark blue was observed, further confirming the formation of V
4+ ions. V
2O
5 is an acidic oxide, which is highly suitable for the adsorption of basic molecules, such as TEA and consequently a larger response of the V
2O
5 hollow spheres sensor to TEA resulted
[36].
Generally, resistive based gas sensors work at high temperatures, which need external heaters and increase the power consumption. Therefore, development of room temperature gas sensors not only solves above problems, but also integration with flexible substrates become easier. Furthermore, possible risk of explosion during sensing of explosive gases such as H
2 gas significantly decreases. Hollow spheres comprising numerous nanocrystals of V
2O
5 as a shell were synthesized by a facile polyol approach for room temperature hydrogen gas sensing. The surface area of hollow spheres was about 356 m
2/g and, therefore, it provided a large active surface area for adsorption of target gases. Furthermore, its porous structure led to further enhancement of gas reactions. Furthermore, the sharp corners as well as the edges of the tiny building blocks of hollow spheres were reported as highly active sites for enhancement of the sensing reactions during hydrogen sensing
[37].
Another room temperature gas sensor was realized from V
2O
5 nanoneedles which were synthesized by a physical vapor deposition method
[38]. The sensor exhibited a response (R
a/R
g) of 2.37 to 140 ppm acetone at room temperature. The relevant reaction was as follows:

The most energetically favorable gas reaction is that a surface oxygen atom attacks the carbonyl carbon to form a C-O bond. Acetone contains the carbonyl group and because of the greater electronegativity of oxygen; a carbonyl group is a polar functional group and, therefore, it has a larger dipole moment (D = 2.88), relative to other tested gases, leading to the higher response of the gas sensor to acetone.
Trimethylamine (TMA; (CH
3)
3N)) is generated from dead fish and, therefore, the concentration of TMA is an indicator of the freshness of fish
[39]. Furthermore, exposure to TMA vapor can cause nausea, headaches, and irritation to the eyes
[40]. In this regard, spherical V
2O
5 hierarchical nanostructures comprised of plenty of nanosheets were produced through a hydrothermal method. The optimal sensor based on spherical V
2O
5 hierarchical nanostructures displayed a response of ~2.8 to 100 ppm TMA along with fast response/recovery times (5/28 s) at 240 °C. The spherical V
2O
5 nanostructures were comprised of numerous monocrystalline nanosheets, and therefore they have a unique three-dimensional hierarchical structure which provided plenty of active sites for gas molecules. Accordingly, the reaction between chemisorbed oxygen ions and TMA molecules, resulted in a decrease in electrical resistance and contributed to the sensor signal.

In addition, in a monocrystalline structure free electrons were able to transfer faster than in a polycrystalline structure, which results in fast response and recovery times of gas sensors and improvement of sensing properties
[41].
Table 1 presents the gas sensing characteristics of pristine V
2O
5 gas sensors, where different morphologies of V
2O
5 prepared using various methods have been successful for sensing of different gases.
Table 1. Gas sensing properties of pristine V2O5 gas sensors.
V2O5 Morphology
|
Synthesis Method
|
Target Gas
|
Conc. (ppm)
|
Response (Ra/Rg) or (Rg/Ra)
|
T
(°C)
|
Response time/Recovery Time(s)
|
Ref.
|
Hollow spheres
Solid spheres
|
Solvothermal
|
C3H9N
|
500
500
|
9.7
3.08
|
370
|
20/83
45/150
|
[36]
|
Hollow spheres
|
Chemical synthesis
|
H2
|
200
|
2.8
|
RT
|
50/10
|
[37]
|
Nanoneedles
|
PVD
|
C3H6O
|
140
|
2.35
|
RT
|
67/-
|
[38]
|
Hierarchical nanostructures
|
Hydrothermal
|
C3H9N
|
10–200
|
1.13-3.57
|
240
|
) of 9 to 100 ppm diethylamine along with good selectivity to diethylamine gas and a fast response time of 2 s at 350 °C
[63]. Improved sensing performance was related to the formation of V
2O
5-Fe
2O
3 heterojunctions and catalytic effect of V
2O
5 to diethylamine.
Porous silicon (PS) is a good candidate for sensing studies due to its offering of a high surface area and a porous structure. In addition it can be simply prepared by a chemical etching process
[64]. Therefore, composites between V
2O
5 and PS can be promising for gas sensing studies. In a relevant study, thin V films were decorated on the PS by sputtering at different times of 30 and 60 min and then, the samples were annealed at 600 °C
[65]. The PS/V
2O
5 NRs structure provided a better response than pristine PS at 25 °C, and the sensor sputtered for 60 min exhibited the highest response of 7.4 to 2 ppm NO
2 gas. Both the PS and V
2O
5 NRs had plenty of dangling bonds, oxygen vacancies and defects, leading to high adsorption of oxygen molecules even at room temperature. In the interfaces between PS and V
2O
5, p-n heterojunctions formed and, upon exposure to NO
2 gas, the significant modulation of electrical resistance in the heterojunctions led to the appearance of a sensing signal.
Graphene and its derivations such as graphene oxide and reduced graphene oxide have high surface areas and unique electrical properties which can be beneficial for gas sensing studies
[66][67]. The initial resistance of the GO is high, limiting its practical applications in pristine form
[68][69]. However, after the reduction GO to RGO, there are some defects, vacancies and functional groups which are useful for gas sensing applications
[70]. In a relevant study, an RGO surface was decorated with Mn
3O
4 and V
2O
5 NOs via a hydrothermal method for detection of H
2 gas at 30 °C. The sensor showed a high response (ΔR/R
a × 100) of 174% to 50 ppm H
2 gas at room temperature. The sheet-like structure of RGO provided a large surface area for gas sensing reactions. In addition, because of the formation of p-n (RGO-V
2O
5) and p-p (RGO-Mn
3O
4) heterojunctions, significant modulation of resistance in the presence of H
2 occurred, resulting in the generation of a sensing signal
[71]. In another study, a V
2O
5 film was prepared by a reactive sputtering technique and then, RGO was decorated over the V
2O
5 thin film by a drop casting method for NO
2 sensing studies. The sensor showed a response of 50.7% to 100 ppm NO
2 gas at 150 °C. However, its recovery time was long (778 s). Formation and modulation of the p-n heterojunction at the interface of RGO and V
2O
5 was the main reason for the detection of NO
2 gas. Moreover, the presence of active sites such as oxygen functional groups on the RGO surface improved the sensing response
[72].
Not only can V
2O
5-decoration be a useful technique to enhance the gas sensing properties, but decoration of other metal oxides or noble metals on the surface of gas sensor can also be a good technique to improve the gas sensing properties of V
2O
5-based gas sensors. A P-type CuO with excellent catalytic activity is extensively used for sensing studies
[73]. The work function of CuO is 5.3 eV, which is different to that of V
2O
5 (4.7 eV). Therefore, when heterojunctions form between the CuO and V
2O
5, enhanced gas response can be expected. In this context, hollow nanostructures using CuO decorated V
2O
5 nano-strings of pearls were fabricated through an electrospinning method. The V
2O
5/CuO sensors demonstrated a response (R
a/R
g ) of 8.8 to 500 ppm acetone at 440 °C, which was more than three times higher than that of bare V
2O
5 NFs. The improved performance was related to the generation of CuO/V
2O
5 p-n heterojunctions, which provided plenty of resistance modulation sources and upon exposure to acetone gas higher response was resulted
[74].
Table 2 shows the gas sensing properties of decorated or doped V
2O
5-based gas sensors, where different synthesis methods along with different morphologies and various materials have been reported to realize gas sensors for the sensing of toxic gases.
Table 2. Gas sensing properties of decorated or doped V2O5-based gas sensors.
Sensor
|
Synthesis Method
|
Target Gas
|
Conc. (ppm)
|
Response (Ra/Rg) or (Rg/Ra)
|
Working Temp. (°C)
|
Response Time/Recovery Time(s)
|
Ref.
|
Pd decorated porous Si/V2O5 nanopillars
|
DC sputtering
|
NO2
|
2
|
4.5
|
RT
|
-/-
|
[59]
|
Ru-decorated layer structure V2O5
|
Hydrothermal
|
NH3
|
130
|
4 *
|
RT
|
~2/~12
|
[62]
|
V2O5-decorated α-Fe2O3 nanorods
|
5 NPs, the surface area was only ∼16 m
2/g with a pore size of 12.9 nm, while the BET surface area of V
2O
5@TiO
2 C-S NPs was greatly increased to ∼151 m
2/g, and the average pore size was ∼6.4 nm. Thus, the significant increase in surface area was an advantage for C-S NPs, which directly affected the gas sensing studies. The C-S sensor showed a response (R
a/R
g) of 8.6 to 100 ppm ammonia at 365 °C. Upon intimate contact between the V
2O
5 and TiO
2, electrons moved from TiO
2 to V
2O
5, resulting in the creation of an electron depletion layer on both sides of the TiO
2 shell layer. Upon injection of NH
3 gas into the gas chamber, the electrons released back caused the narrowing of the electron depletion layers which finally modulated the electrical resistances of the gas sensor. Moreover, based on DFT calculations, the NH
3 molecule showed the lowest adsorption energy (−1.04 eV) on the anatase TiO
2 (101) surface, which explained the higher selectivity of the gas sensor to NH
3 among other tested gases. The optimal sensing temperature of the gas sensor was registered at 365 °C. The reactions on the surface of TiO
2 can be shown as follows:
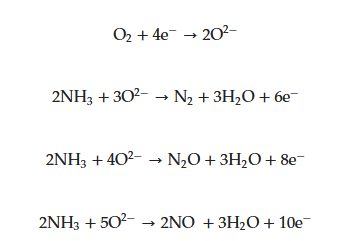
Thus, more electrons can be released when N
xO (x = 1, 2), demonstrating the high response toward NH
3 at elevated temperatures (>300 °C).
Table 3 exhibits the sensing properties of various V
2O
5-based composite gas sensors, where different materials along with various morphologies have been used for sensing of different gases.
Table 3. Gas sensing properties of V2O5-based composite gas sensors.
Sensing Material
|
Synthesis Method
|
Target Gas
|
Conc. (Ppm)
|
Response (Ra/Rg) Or (Rg/Ra)
|
T (°C)
|
Response Time/Recovery Time(S)
|
Ref.
|
V2O5/In2O3 core–shells
|
Hydrothermal
|
n-propylamine
|
200
|
4
|
190
|
48/121
|
[86]
|
MoO3-V2O5 thin films
|
Chemical spray pyrolysis
|
NO2
|
120
|
80 *
|
200
|
118/1182
|
[87]
|
|
(MoO3)0.4(V2O5)0.6 sheet composite
| Electrospinning
|
Chemical spray pyrolysis
C4H11N
|
100
300
|
115
9
|
350
|
2/40
|
[63]
|
|
| 39/453 |
|
| [88]
|
V2O5 decorated SnO2 NWs
|
Au/V2O5/CuWO |
VLS/ALD
|
4 composite
NO |
Hydrothermal
2
|
NH3
200 ppb
|
|
5
3.6
|
250
5/28
|
|
-/-
[41]
|
| [ | 75 | ] |
|
| 2.7 |
|
| 150 |
|
35/33
|
[89]
|
Flower-like
|
|
Porous Si/V2O5
NR composite
Hydrothermal
|
Galvanostatic electrochemical etching
5
|
NO2
2.25
|
|
2
200
|
7.4
13/13
|
RT
[42]
|
| -/- |
|
| [ | 65 | ] |
|
90]
|
Nanorods
|
rGO/Mn3O4/V2O5 nanocomposite
|
CVD
|
|
V2O5/polyvinyl acetate NF composite |
Hydrothermal
| NH3
|
H
100
|
2
235 *
|
400
|
-/-
|
|
50
[43]
|
|
Electrospinning
|
| 175 |
|
RT
|
NH3
|
0.8
|
6 *
|
26082/92
|
|
-/-
[ |
[91]
|
Nanorods
|
Solvothermal
|
V2O5/ZnV2O6 nanobelt composite
|
|
Chemical route
C2H5OH
NH3
|
C
500
|
1.04
1.02
|
2
RT
|
H5 |
-/-
|
[44]
|
71 | ] |
| OH
|
2000
|
16.5
|
240
|
Spherical
|
Precipitation
|
C2H5OH
NH3
|
1.04
1.06
|
-/-
|
[45]
|
Flower-like
|
Hydrothermal
|
1-butylamine
|
100
|
2.6
|
140
|
9/49
|
[46]
|
Nanofibers |
Pd-decorated CuO NWs
|
UV irradiation
|
H2S
|
100
|
1.962
|
100
|
-/-
|
[72]
|
| -/- |
|
| [ | 92 | ] |
|
V2O5/CuO nano-string of pearls
|
|
TiO2/V2O5 NF composite
| Electrospinning
|
Electrospinning
C3H6O
|
500
|
9
|
440
|
~40/~100
|
|
100
|
24.6
|
350
|
6/7
|
[93]
[74]
|
CuO-decorated V2O5 NWs
|
ZnO/V2O |
Hydrothermal and wet-deposition
|
H2S
|
5
23
|
thin films
31.86
|
Spray pyrolysis
|
C7H8
|
400
|
2.3
220
|
27
|
23/28
|
[94]
|
|
Electrospinning
|
9.5
|
500 *
|
RT
|
-/-
|
RT
[47]
|
| -/- |
|
| [ | 77 | ] |
|
|
Fe2O3 activated V2O5 nanotubes
|
Hydrolysis
|
C2H5OH
|
SnO2/V2O5 composite
|
Sol-gel
|
C6H6
|
200
|
10.5
|
275
|
-/-
|
[
130/218
|
[76]
|
Flower-like Sheet-like
|
SnO2 NP-decorated V2O5 NWs
|
Hydrothermal
|
C2H5OH
|
1000
|
1.3
Hydrothermal
|
100
|
3.6
2.8
|
300
|
1000
25/14
17/14
|
[ |
2.2
48 |
270
]
|
| -/- |
|
| [ | 78 | ] |
|
Nanofibers
|
|
TiO2-decorated V2 | Sol–gel
|
O5 NWs
|
Hydrothermal
NH3
|
2.1 |
O3
|
1.25
|
11 *
|
2.6 *
200
|
300
50/350
|
|
~180/~180
[49]
|
| [ | 79 | ] |
|
Flower-like
|
DC sputtering
|
CH4
|
500
|
100
|
206/247
|
[50]
|
RGO-decorated V2O5 thin film
|
Reactive sputtering and drop casting
|
NO2
|
100
|
50.7
|
150
|
-/-
|
[72]
|
Hydrothermal
|
Au NP-decorated V2O5
|
C8H10
|
3
|
Two-step in-situ reduction of Au and thermal oxidization as V2O5
|
Amines
300
|
100
44/74
|
7.5
[51]
|
| 240 |
|
| 90/35 |
|
| [80]
|
Nano stars
|
Hydrothermal
|
He
|
300
|
53 *
|
RT
|
9/10
|
[52]
|
Nanorods
|
Chemical spray pyrolysis
|
NO2
|
100
|
24.2 *
|
200
|
13/140
|
[53]
|
Nanofibers
|
Chemical spray pyrolysis
|
C8H10
|
Pd-decorated V2O5 thin film
|
DC magnetron reactive sputtering
|
H2
|
100
|
5.7
|
100
|
~6/14.8
|
[81]
|
V2O5-
doped SnO2 NFs
|
Electrospinning
|
C |
27
|
RT
|
80/50
|
[54]
|
6 | H | 6
|
25
|
6.32
|
325
|
3/47
|
[82]
|
Nanowires
|
Melt quenching
|
C2H5OH
|
1000
|
9.09
|
330
|
-/-
|
[55]
|
Thin film
|
Plasma focus method
|
H2
|
50 *
|
275
|
-/-
|
[56]
|
Chemical spray pyrolysis
|
NO2
|
100
|
41 *
|
200
|
20/150
|
[57]
|
* Response = Δ𝑅/𝑅𝑎×100; RT: Room temperature; PVD: Physical vapor deposition; CVD: Chemical vapor deposition.
3. Decorated/Doped V2O5 Gas Sensors
Based on the above section about pristine V
2O
5 gas sensors, pristine V
2O
5 nanostructures suffer from low sensitivity and selectivity, which hinder their applications for sensing applications
[58]. Accordingly, different strategies, such as p-n heterojunction formation
[59], n-n heterojunction formation
[60][61] and noble metal decoration
[62] have been proposed to enhance their sensing properties.
V
2O
5 decoration is a good strategy to enhance the sensing properties of gas sensors. V
2O
5-decorated α-Fe
2O
3 composite NRs were prepared by an electrospinning technique and they had a high surface area of 30.5 m
2/g. The composite exhibited a response (R
a/R
g
* Response = Δ𝑅/𝑅𝑎×100; RT: Room temperature; VLS: Vapor-liquid-solid; NR; Nanorod; NP; Nanoparticle; NF; Nanofiber.
4. Nanocomposites/Nanohybrids of V2O5 Gas Sensors
Core-shell (C-S) nanocomposites are among the most promising structures for gas sensing studies, as in these structures the interfaces between two different materials are maximized, resulting in significant modulation of electrical resistance upon exposure to target gases
[83][84][85]. Fu et al.
[39], synthesized V
2O
5-TiO
2 core-shell (C-S) NPs for NH
3 studies. For pristine V
2O