2. Metal Oxide-Based Gas Sensors: Introduction, Design, and General Mechanism
Metal oxide-based gas sensors are often fabricated by coating a sensing layer over an insulating substrate and then providing it with interdigitated electrodes
[44][54]. A heater is also included in many sensors in order to raise the temperature of the gas sensor up to 500 °C
[45][55]. Micromachined gas sensors can be used for attaining high temperatures
[46][56]. Furthermore, they are fabricated from nanoscale materials, which have a high surface area and high reactivity to target gases
[47][48][57,58]. In addition, it is well known that morphology is one of the most important factors affecting the gas sensing performance. Therefore, different morphologies, such as nanoparticles
[49][59], nanorods
[39], hollow spheres
[50][60], nanowires (NWs)
[51][61], hierarchical
[52][62], porous spheres
[53][63], flowers
[54][64], and dumbbell-like
[55][65] morphologies have been reported for acetone or other gas sensing studies.
The general mechanism of sensing for metal oxide-based acetone gas sensors is as follows. Initially, in air, due to high electron affinity of oxygen molecules (0.43 eV
[56][66]), they get adsorbed on the surface of the sensor and take electrons from the sensing layer. Accordingly, depending on the operating temperature, different oxygen ion species will be available on the surface of gas sensors as demonstrated by the following equations
[57][67]:
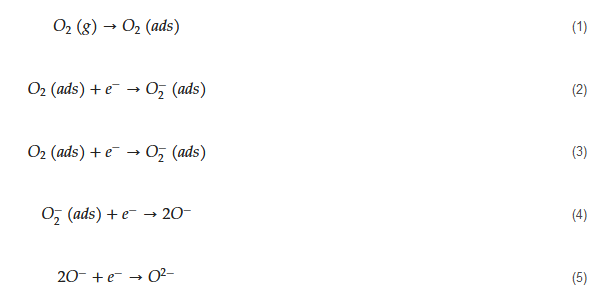
The tested gas species depend on the sensing material and sensing temperature. However, generally, molecular ions are stable below 150 °C, while other forms are stable at higher temperatures
[58][59][68,69]. Due to abstraction of electrons and depending on the n-type or p-type nature of gas sensor, an electron depletion layer or a hole accumulation layer, respectively, will be generated on the surface of gas sensor. Since the majority of charge carriers in n-type sensors are electrons and those in p-type gas sensors are holes, the resistance of n-type sensors increases relative to vacuum, while the resistance of p-type gas sensors decreases relative to vacuum. When acetone gas is injected into a gas test chamber, it will react with oxygen ions that were already adsorbed and electrons will be released as follows
[60][61][70,71]:

The released electrons are injected in to the sensing layer and the width of electron depletion layer of an n-type sensor or hole accumulation layer of a p-type sensor is decreased. Accordingly, the resistance of n-type sensors decreases and that of p-type gas sensors increases upon exposure to acetone. The response of gas sensor to acetone gas, which is a reducing gas, is usually defined as R
a/R
g for n-type gas sensors and R
g/R
a for p-type gas sensors, where R
a is the resistance of gas sensor in the presence of air and R
g is the resistance of gas sensor in the presence of acetone gas.
Figure 2 shows the general acetone sensing mechanism for pristine n-type and p-type metal oxides. For noble metal-decorated or heterojunction metal oxide-based gas sensors, in addition to above mechanism, formation of Schottky contacts, formation of n–n, n–p, or p–p heterojunctions, and catalytic effects of noble metals are other mechanisms which can further enhance the response of gas sensor and will be explained in the subsequent sections of this
article
ntry.
Figure 2.
Schematic illustration of acetone sensing mechanism in (
a
) n-type metal oxides and (
b
) p-type metal oxides.
In general, nanostructured materials such as 0-dimensional, 1-dimensional, and 2-dimensional metal oxides have higher sensitivity relative to their bulk counterparts. In fact, such materials not only have a high surface area which greatly enhances the response of gas sensors due to offering of more adsorption sites for the target gas molecules but they also have many contact points which act as potential sources for the resistance modulation. Another advantage of nanostructured metal oxides stems is from the fact that when the size of materials reaches to the Debye length (
λD), adsorption of target gas molecules on the surface of sensor leads to great modulation of electrical resistance, leading to the appearance of a high sensing signal
[62][72].
3. Pristine Acetone Gas Sensors
In 1991, bismuth ferrite (BiFeO
3) nanoparticles (NPs) were studied for sensing applications and it was reported that sensing performance was related to the chemical composition
[63][73]. Moreover, recently, BiFeO
3 NPs were used for acetone sensing and they showed a response (R
g/R
a) of 10–12 ppm acetone gas at 350 °C with a fast response time of 25 s and a recovery time of 17 s
[64][74]. In another study, p-type praseodymium ferrite (PrFeO
3) was used for sensing studies. Electrospun hollow PrFeO
3 nanofibers with a high surface area of 33.74 m
2/g showed a high response (R
g/R
a) of 141–200 ppm acetone at 180 °C
[65][75].
Semiconducting spinel ferrites (AFe
2O
4), in which the transition metal cation “A” was included into the lattice of the parent structure of (Fe
2+Fe
23+O
4)
[66][67][68][69][76,77,78,79], had environmental friendliness, low cost, and excellent stability
[70][71][72][80,81,82]. Therefore, they were studied for acetone sensing applications
[73][74][83,84]. For example, super fine porous NiFe
2O
4 spheres were fabricated using a solvothermal method. The surface area of the product was 20.04 m
2/g with an average pore size of ~8.9 nm. The fabricated sensor revealed a high response (R
g/R
a) of 27.4–100 ppm acetone with a fast response time of 2 s at 250 °C. The sensor showed a moderate selectivity to acetone. In addition, the response to acetone was significantly decreased with an increase of relative humidity, which limits its real-world applications
[18].
4. Doped Acetone Gas Sensors
4.1. Binary Metal Oxide Gas Sensors
Doping is a widely used strategy to enhance acetone sensing properties
[75][76][99,100]. In an interesting study, the bare and W-doped NiO hierarchical hollow spheres were synthesized by a hydrothermal method. The sensor with 4.0 at% of W-doping showed a response (R
g/R
a) of 198.1–100 ppm acetone at 250 °C, while the response of bare sensor was 139 times lower. Higher response of doped sensor was related to the lower crystalline size as a result of W-doping, higher surface area (217.2 m
2 g
−1), and higher concentrations of hole carriers due to W-doping, which created additional holes in the NiO
[77][101].
2D Sn-doped ZnO ultrathin nanosheet networks were synthesized by a hydrothermal method
[78][102]. At 320 °C, which was reported as the optimal sensing temperature, the sensor showed a response (R
a/R
g) of 5.6–50 ppm acetone gas. Role of Sn-doping was attributed to the creation of more defect in crystal by substitution in ZnO crystal structure.
La
2O
3 is rarely used for acetone sensing studies. Xu et al.
[79][103] synthesized bead-like 1 wt% La
2O
3-doped ZnO NFs through electrospinning for acetone sensing studies. The gas sensor showed a response (R
a/R
g) of 48–100 ppm acetone gas at 340 °C. The excellent gas sensing properties caused by La doping were related to the larger surface area of gas sensor, the increased adsorption capacity of O due to presence of La
2O
3, and the useful hetero contacts between n-type ZnO and p-type La
2O
3.
Co-doped ZnO NFs were produced through an electrospinning process followed by an annealing process
[80][104]. At optimal sensing temperature of 360 °C, the response (R
a/R
g) of 0.5 wt% Co-doped ZnO NFs to 100 ppm acetone was ~16, while that of pristine sensor was only 4.4. Additionally, response and recovery times of optimal sensor were 4 and 6 s, respectively. High response to acetone was due to the high surface area of synthesized NFs and presence of plenty of NF–NF junctions in the netlike structure. Furthermore, Co-doped sensor showed a decreased NF diameter relative to pristine ZnO NFs. Finally, catalytic effect of Co
3O
4 led to easy oxidation of acetone, contributing to the sensor signal.
Hollow NFs can provide significantly higher surface area relative to NFs and therefore, it can be expected to have high response to acetone gas. Y-doped SnO
2 hollow NFs were synthesized through electrospinning technique. The 0.4 wt% Y-doped SnO
2 hollow NFs showed a response (R
a/R
g) of 174–500 ppm acetone gas at 300 °C
[81][105]. High sensing performance to acetone gas was related to the high surface area of gas sensor (29.46 m
2/g), presence of many small nanograin–nanograin junctions on the surface of SnO
2 hollow NFs, and catalytic effect of surface “Y” clusters, which enhanced gas response to acetone gas.
Like other rare earth elements, Eu rarely has been studied for gas sensing studies. In a study by Jiang et al.
[82][106], Eu-doped SnO
2 NFs were produced by electrospinning for acetone sensing studies. The sensor was able to detect even 0.3 ppm acetone, making it a potential candidate for the breath diagnosis of diabetes. When Eu
3+ ions diffused into the SnO
2 lattice and substituted Sn
4+ ions, the mismatch in ionic radius between Eu
3+ (0.947 Å) and Sn
4+ (0.69 Å) resulted in the lattice distortion and defects, leading to enhanced gas response. Furthermore, Eu
2O
3 was able to accelerate the reactions between acetone molecules and absorbed oxygen species to release electrons to the conduction band, leading to an improved gas response.
4.2. Ternary Metal Oxide Gas Sensors
P-type LaMnO
3+δ (LMO) perovskites oxides have fascinating properties owing to excess oxygen as well as coexistence of Mn
3+/Mn
4+ ions with different oxidation states
[83][116]. In order to enhance gas sensing properties of the LMO gas sensor, yttrium-doped LMO NPs (namely, La
0.85Y
0.25MnO
3+δ NPs) were synthesized via a sol–gel method. It showed a response (R
g/R
a) of ~26–500 ppm acetone gas at 300 °C. However, its response to ethanol was almost 5 times lower than that of the pristine gas sensor. Due to doping of Y
3+ and in order to maintain the charge neutrality, the Mn
4+/Mn
3+ ratio was increased, and accordingly, more oxygen species were adsorbed on the surface of gas sensor. Due to the p-type nature of gas sensor, initial resistance of the sensing layer was significantly decreased, and upon exposure of sensor to acetone vapor, the resistance significantly increased, leading to high response of sensing layer to acetone
[84][117].
P-type ytterbium ferrites, with a general formula of YbFeO
3, have high stability, and their properties can be tuned through metal doping. In an interesting study related to YbFeO
3, 20 at% Ca-doped YbFeO
3 (Yb
0.8Ca
0.2FeO
3) was synthesized via a sol–gel process for sensing study. Interestingly, the response to acetone gas was increased in humid air at room temperature. The presence of –OH groups on the surface of the sensor was reported to be the reason for such an increase. However, it seems that more studies are needed to find such a strange behavior of this gas sensor
[85][118].
5. Decorated/Loaded Acetone Gas Sensors
SnO
2, is a metal oxide n-type semiconductor (Eg = 3.6 eV) with high stability, low synthesis costs, and high sensing properties due to its high mobility of electrons (160 cm
2/V·s)
[86][87][119,120]. SnO
2 hierarchical structures loaded with Sm
2O
3 (0.5, 1, 2.5, and 4 mol%) were synthesized by a hydrothermal method and subsequent isometric impregnation technique. It was revealed that the 2.5 mol% Sm
2O
3/SnO
2 gas sensor showed a response (R
a/R
g) of 41.14–100 ppm acetone gas at 200 °C, with a limit of detection of 100 ppb. Upon replacement of Sm
3+ in SnO
2 lattice, oxygen vacancies were formed and much higher target gases were adsorbed on the surface of the gas sensor. Furthermore, the catalytic effect of Sm
2O
3 was reported to be important for acetone sensing
[88][121].
TiO
2 NPs were decorated on In
2O
3 NWs for acetone sensing studies
[89][123]. At 250 °C, the fabricated sensor showed a response (R
a/R
g) of ~34–10 ppm acetone which was higher than that of ethanol and other interfering gases. Enhanced sensing response to acetone was due to the changes in the surface depletion layer width and potential energy barrier width of In
2O
3 NWs.
Rh
2O
3-decorated WO
3 NFs were synthesized via an electrospinning process for acetone sensing studies
[90][124]. The sensor showed a response (R
a/R
g) of 41.2–5 ppm acetone gas at 300 °C. When Rh
2O
3-decorated WO
3 NFs were exposed to target gas, due to reduction of Rh
2O
3 NPs, the width of depletion region inside of WO
3 was decreased, leading to enhanced gas response towards acetone. Furthermore, presence of oxygen vacancies contributed to generation of a high sensing signal.
Not only metal oxides but also noble metals can be decorated on the surface of sensing layers. Different noble metals have been decorated on the sensing layer for acetone sensing studies
[91][92][125,126]. Hollow porous Fe
2O
3 nanocubes were synthesized, and subsequently, Pt NPs were loaded on the surface of Fe
2O
3 nanocubes by means of a reduction process. The sensor had a response (R
a/R
g) of 25.7–100 ppm of acetone at 139 °C. Both chemical sensitizations and electronic sensitization of Pt had a high contribution to the sensing signal. Oxygen molecules were dissociated on the surface of Pt and subsequently spilt over the surface of Fe
2O
3, leading to high adsorption of oxygen ions. The surface of Pt NPs preferentially adsorbs oxygen molecules in air and then spill the oxygen species over to the Fe
2O
3, owing to the catalytic promotion of Pt. Furthermore, the electrons were transferred from Fe
2O
3 to Pt, and Schottky barriers were formed in air. In an acetone atmosphere, the height of the Schottky barrier significantly decreased, leading to an enhanced response to acetone. Furthermore, it was reported that in air, some PtO
x can be formed and the resultant p–n heterojunctions can enhance resistance modulation in the presence of air
[93][127].
6. Composite Acetone Gas Sensors
In the literature, there are many studies related to acetone sensing properties of composite materials. This is due to the fact that in composite materials, plenty of heterojunctions can form, leading to significant modulation of electrical resistance
[54][64]. However,
here,in th
e researchersis review paper, we just report the results of the most interesting acetone gas sensors in terms of design or gas sensing performance.
Electrospun Pt@In
2O
3 core–shell composite NWs were prepared for acetone sensing studies
[94][129]. The sensor showed a highly improved response, short response and recovery time of 14 and 16 s, respectively, for 1 ppm of acetone, and high selectivity and stability compared with a sensor based on pristine In
2O
3 NWs due to the increase in surface resistance and the presence of heterojunctions. In addition, detection limit was low as 10 ppb, which was much lower than the concentration level of 1.8 ppm in the exhaled breath of diabetic patients. The influence of the large amount of moisture was greatly weakened by using the molecular sieve as a moisture filter layer, leading to much improved sensitivity to acetone in clinical sample detection.
WO
x≤3 oxide has different nonstoichiometric phases, namely, WO
2, WO
2.72, WO
2.8, and WO
2.9. In general, these phases have a lot of oxygen vacancies, which promote the adsorption of target gases on the surface of gas WO
x≤3-based gas sensors
[95][130]. In addition, MXenes are a new class of 2D transition metal of carbides/nitrides, that are rich with functional groups such as –O, –OH, and –F, which are beneficial for gas sensing studies
[96][131]. In this regards, a series of WO
2.72(W
18O
49)/Ti
3C
2T
x composites were solvothermally prepared for sensing studies towards acetone
[97][132]. The W
18O
49 nanorods were distributed on the surface of Ti
3C
2T
x nanosheets, leading to an increase of adsorption sites relative to pristine sensors. The W
18O
49/Ti
3C
2T
x–2% sensor showed a response (R
a/R
g) of 4.2–5 ppm acetone gas at 300 °C, which was higher than other gas sensors with different amounts of Ti
3C
2T
x as well as pristine gas sensors. First, due to presence of functional groups on the surface of Ti
3C
2T
x, the acetone molecules were effectively adsorbed on the surface of the gas sensor. Second, due to metallic nature of for the Ti
3C
2T
x, heterojunctions were creased on the interfaces between Ti
3C
2T
x and W
18O
49, and in the acetone atmosphere, the modulation of heterojunction potential barrier led to enhanced sensing response. Third, for the compositions with a higher amount of Ti
3C
2T
x, some –F groups were attached to the surface, leading to decrease of gas response. Finally, too much Ti
3C
2T
x caused the stacking of the nanosheets, and this decreased the adsorption sites of gas sensor.
CuO with p-type sensing properties has been used for sensing studies due to its low synthesis cost and catalytic effects
[98][133]. A very low power consumption gas sensor was prepared using Fe
2O
3/CuO-based nanostructures which were fabricated by direct ink writing (DIW) on top of the surface of an insulating (glass) substrate. Due to the presence of copper oxides and iron oxides, many heterojunctions were produced on the surface of glass substrate. The power consumption of gas sensor at 300 °C was only 0.26 μW, and the gas sensor showed a response of 50% to 100 ppm acetone vapor. Formation of Fe
2O
3/CuO heterojunctions in air and subsequent modulation of potential barriers in acetone vapor were the main reasons for acetone sensing mechanism
[99][134].
In comparison to the dense and thick films, three-dimensional (3D) ordered composites have a large surface area, which guarantees the high number of adsorption sites, leading to higher sensing properties. Moreover, because of having a porous structure and open channels, surrounding gases can diffuse into the deep part of gas sensor, contributing to high gas response. In this context, the 3D inverse opal (3DIO) composite of ZnO-Fe
3O
4 was prepared using a template method
[100][135]. The ratio of Fe/Zn atoms was varied to find the optimal gas sensor. For the gas sensor with a Fe:Zn atom ratio of 2:10 at 485 °C, a response (R
a/R
g) of 47–50 ppm acetone vapor was reported. It was also reported that the amount and size of mesoporous increased with the increase of Fe. As a result, the surface area increased and more gas molecules were able to be adsorbed on the surface of sensor. However, for the sensor with Fe:Zn, atomic ratio of 3:10, the structure was damaged and sensing properties decreased. Furthermore, ZnO/Fe
2O
3 heterojunctions significantly enhanced the sensing properties. ZnO/Fe
2O
3 heterojunctions were initially formed in air upon intimate contact between ZnO and Fe2O3. Subsequently, by exposure of the gas sensor to acetone vapor, the height of heterojunction barriers was decreased, leading to modulation of sensor resistance. This ultimately contributed to the gas response.
The two-dimensional (2D) heterostructure of the C
3N
4-SnO
2 nanocomposite sensors with a high surface area of 57.13 m
2/g were prepared for gas sensing studies
[101][136]. The sensor showed a response (V
g/V
a) of 29–100 ppm acetone gas at a sensing temperature of 380 °C with fast response/recovery times (7 and 8 s, respectively). In addition, it was possible to detect as low as 67 ppb acetone, which is way below the exhaled breath concentration of diabetic people. Excellent acetone sensing properties were related to the exchange of electrons from SnO
2 to C
3N
4 and formation of heterojunctions. Furthermore, the large surface area of C
3N
4 layer provided high number of adsorption sites for target gases. In addition, good selectivity of gas sensor was related to the large dipole moment of acetone arising from the C–C=O group of acetone. Dipole moment of acetone was higher than that of ethanol (1.69 D), methanol (1.70 D), NO (0.159 D), NO
2 (0.316 D), NH
3 (1.471 D), and CO (0.112 D) gases
[102][113], leading to more interaction between acetone and the sensing layer.
NiO/ZnO composites were prepared by decoration of NiO NPs on the surfaces of ZnO hollow spheres using a solvothermal technique. The response (R
a/R
g) of NiO/ZnO composite sensor to 100 ppm acetone was ~29 at 275 °C, and both response and recovery times were 1 and 20 s, respectively. In dry air, the p–n junction between n-type ZnO and p-type NiO was produced. As a result, the resistance of the sensor was increased in air. In the acetone atmosphere, due to release of electrons to the surface of gas sensor, the resistance significantly decreased and a response appeared.
Defects and functional groups presenting on the graphene oxide (GO) surface act as high-energy adsorption sites for the gas molecules and can increase the response to acetone gas. In this regards, ZnO nanosheet/GO nanocomposites were prepared for detection of acetone
[103][92]. The sensor with 10 wt% GO showed the highest sensing properties and a response (R
a/R
g) of 35.8–100 ppm acetone was observed at 240 °C. Presence of p-GO and n-ZnO potential barriers, high surface area of 2D nanocomposite, and presence of functional groups on the surface of GO were the main factors contributing to the sensor signal.
Organic–inorganic hybrid materials generally have enhanced sensing properties such as operating temperature and response time relative to pure materials due to a synergic effects between the different materials
[104][138]. A polyaniline (PANI)/SnO
2 hybrid material was prepared by a hydrothermal method for acetone sensing studies. The sensor revealed the highest response (R
g/R
a) of 1.68–800 ppm acetone gas at 60 °C. PANI is a p-type semiconductor and SnO
2 an n-type, so that in intimate contacts, formation of p–n heterojunctions can create potential barriers in air. In acetone atmosphere, the height of potential barriers decreases, leading to sensing signal. However, the response was not compared with metal oxide nanocomposites
[105][139].
Metal-organic frameworks-derived zinc oxide nanopolyhedra/S, N: graphene quantum dots/polyaniline (ZnO/S, N: GQDs/PANI) nanohybrid was synthesized by in situ polymerization route for acetone sensing applications. The sensor was able to work at room temperature and a response (R
a/R
g) of 1.33–0.5 ppm acetone with a fast response time of 15 s was reported. The presence of PANI in the nanohybrid led to the formation of p–n heterojunctions and also caused to a redistribution of charge carriers at the interface of n-type ZnO and p-type PANI/S, N: GQDs, decreasing the activation energy needed for the adsorption of acetone gas molecules. The presence of S, N: GQDs in the nanohybrid created Schottky contacts, which further enhanced the sensor response through effective capture and migration of electrons
[106][140].
PPy-WO
3 hybrid nanocomposites with different weight percentages of WO
3 NPs (5–40 wt%) dispersed in polypyrrole (PPy) matrix were prepared for acetone sensing studies. PPy-WO
3(20 wt%) sensor showed a fast, fairly sensitive, selective, and enhanced response toward acetone at 90 °C. The enhancement of acetone sensing properties of the PPy-WO
3 hybrid nanocomposite film was related to the effective role of WO
3 NPs in PPy matrix and formation of p–n hetrojunction region. The results demonstrate the potential application of PPy-WO
3 hybrid sensor for noninvasive detection of acetone in breath
[107][141].