Similar to wood, bone is also an anisotropic heterogeneous composite material, as it consists of about 60% inorganic material (calcium and phosphate in a form of natural or calcium-deficient hydroxyapatite), 30% organic material (collagen) and 10% water
[27][28][29]. Similar to wood, which fulfills the function of support in a tree, the main function of bone is to support the static and movement functions of the body. Bone also acts as storage for minerals such as calcium and phosphate, as well as in the maintenance of homeostasis
[30]. Three parts of bone can be observed in the cross-section—cortical bone, also called dense or compact bone, trabecular bone, also called spongy or cancellous bone, and bone marrow cavity. Bone tissue contains three main cell types: osteoblasts, osteoclasts, and osteocytes. Osteoblasts are responsible for bone formation, while osteoclasts are cells that resorb bone. Bone homeostasis is maintained by the connection between bone formation and bone resorption (bone turnover). Osteocytes are cells that are found in fully formed bone and make up most of the bone
[31]. Similarities between wood and bone have been observed and were described as early as the invention of the microscopic magnification itself. The pioneer of microscopy, Antonie van Leeuwenhoek, described the analogy between the osteoid bone structure and the fibre structure of wood
[32]. Since then, several authors have continued researching similarities between wood and bone, revealing the hierarchical macroscopic and microscopic structures of both, as well as functional similarities such as biomechanical characteristics, remodeling, and liquid transportation abilities
[33][34][35][36][37].
Figure 1 shows the structural similarity between cortical bone and wood at the micro and nano level.
3. Wood Species for Bone Implants
Individual species of trees have been studied as a source of biomaterial. Most of the published studies have main purpose of creating biomaterials for bone defect substitution and rarely for creating wood-based orthopedic implants.
3.1. Birch
One of the first studies regarding wood as a possible implant material was performed by Kristen, Bösch et al. using birch wood
[41]. Birch is one of the most widespread and economically important species of deciduous trees in Europe and Scandinavia. Silver birch (
Betula pendula) and European white birch (
Betula pubescens) are among the most common birch species found in most of Europe, up to Central Siberia.
Betula pubescens, which is the northernmost tree species, is more common in the Northern and Eastern parts of Europe.
Betula pendula is more common in the southern regions of Europe, such as the Iberian Peninsula, southern Italy, and Greece
[42]. Birch wood has an average density of 600–650 kg/m
3 and a high Jank hardness of 4000–5000 N, and contains little extractive material, which makes this wood well suited for bone implants
[43][44]. The early studies were conducted in vivo using rabbits. Birch implants were pre-treated with ethanol and placed transcortically into rabbit tibias. Evaluation was done after 3, 5, 14, and 32 weeks. Although the tissues produced a foreign body reaction, a new bone formed around the wood implants. Additionally, bone ingrowth into the implant’s pores was recorded
[45]. Similar ethanol pre-treated birch implants were implanted into a rabbit’s soft tissues. After controls within 2, 6, 12, and 30 weeks, it was concluded that ethanol pre-treatment was not sufficient to prevent a foreign body reaction
[41]. Years later, Rekola, Aho et al. published a novel wood pre-treatment method—preheating of birch implants at different temperatures—140 °C, 200 °C, and 220 °C for 2 h. The implants were placed into the drilled cavities of rabbit femurs and observed after 4, 8 and 20 weeks detecting the bone ingrowth. Preheated birch implants showed better osteoconductivity compared to untreated implants. However, when applying the highest temperature of 220 °C, the biomechanical characteristics of the implants were decreased
[46]. In vitro studies were performed by immersing the birch implants into simulated body fluid (SBF) for 63 days at 37 °C. It was documented that immersion in the SBF significantly decreases the biomechanical properties of the untreated implants, while heat pre-treated implants preserve these properties
[47][48].
3.2. Ash
Ash is a tree of the olive family that is widespread in Europe, Asia, Canada, and North America. As a hardwood with a low content of extractive substances, a high density of 600–680 kg/m
3, strength, and flexibility, it is suitable for bone implant materials
[49]. The in vivo study with ash implants was conducted simultaneously with early birch studies. Ash specimens were ethanol-pretreated and fixed in rabbit calcaneus bones with Achilles tendons reattached and analyzed after 5 and 14 weeks. The ethanol pre-treatment of ash resulted not only in bone ingrowth, but the tendons’ tissues grew into the wood pores as well, along with moderate foreign body reaction
[50].
3.3. Lime, Willow, and Fir
Spruce wood is widespread in Scandinavia, Northern Eastern Europe, North America, Canada, and Japan, and is one of the most economically important coniferous wood species
[51]. The white willow (
Salix alba) is the most well-known of the willows, widely distributed throughout Europe except for the most northern regions. The northern part of Europe, where willow is common, includes the British Isles, the Netherlands, and the Baltic coast (Latvia and Lithuania). Willow is also found in Mediterranean regions, as well as in North Africa (Morocco and Algeria)
[52]. Lime trees are common in Eastern North America (
Tilia americana) and Europe (
Tilia Europen; hybrid wood). All named wood species have a low density—400–450 kg/m
3 for fir and willow, 450–550 kg/m
3 for lime wood. These wood species, together with birch and ash, were used to fix fractures in rabbit femurs by Horsky, Huraj, Paukovic. Implants were untreated before implanting in vivo. Birch, ash, and fir were well tolerated, while lime and willow caused acute inflammatory reaction, indicating that differences in wood species meant that not all species would be suitable for bone implants
[53]. Although all the mentioned wood species contain a high extractive content, they differ in their composition. Fir extract contains the most lignans
[54], while willow extracts contain a large amount of salicylic compounds, flavonoids, and tannins. These substances are bioactive compounds characterized by antipyretic, analgesic, anti-inflammatory, antirheumatic, and anticoagulant properties. As with all bioactive substances, they can be toxic at certain levels
[55][56].
3.4. Juniper
Juniper is the world’s most widespread and northernmost coniferous tree. It is common both in Europe and Asia, as well as in North America and Japan. Juniper can be found both in the farthest North areas of Scandinavia and in the mountain areas of the warmer regions of Southern Europe. The density of juniper is 450–600 kg/m
3 [57]. Juniper has long been studied for its antibacterial properties, but not for use in bone implants. The essential oils in juniper wood can also be toxic at high dosages; therefore, pre-treatment is required
[58]. A unique in vivo study considered juniper wood for potential orthopedic hardware. Hip prostheses were crafted and pre-treated in boiling water for 10 min. The proximal part of rabbit femurs were resected and hemiarthroplasty with the juniper prostheses was performed. Rabbits were allowed to bear weight with no restrictions. Histological analysis was done after 3, 6, 18, and 36 months. No foreign body reaction was documented in any specimens. Initial bone ingrowth was detected after 6 months. After 3 years, wood implants were fully integrated with bone tissues (
Figure 2). Essential oils from juniper were tested for their capacity to induce a toxic response in rats and was demonstrated to be well tolerated, especially when released slowly
[59]. Almost 20 years later, preliminary studies have been carried out for the possible development of bone implants from partially delignified and compressed solid juniper wood, thus improving the mechanical properties of the implant. A compressed wood density of 1170 kg/m
3 was achieved (100% increase compared to natural juniper wood). The modulus of rupture was increased by 85%, reaching 174 MPa, and the modulus of elasticity by 620%, reaching 12,500 MPa
[60].
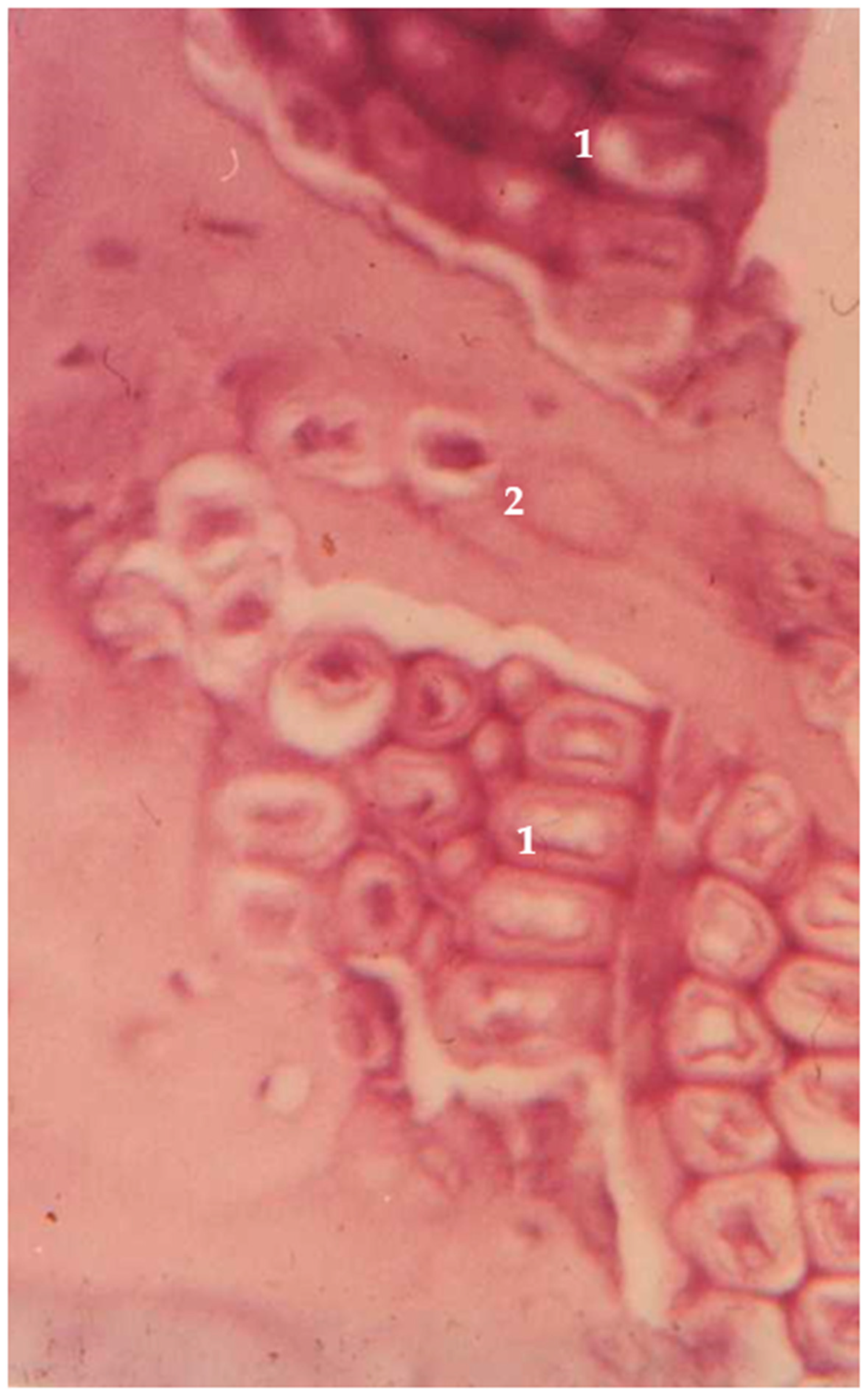
Figure 2. Juniper implant in the in vivo model. 1—juniper implant; 2—bone tissue ingrowth.
Another trial for the development of bone implants has been proposed by pre-treating wood at high temperatures to create a charcoal-type material. In one of the earliest studies, wood from clematis was carbonized at 850 °C for 5 h. Samples were implanted in vivo into rabbit bone, whose tissue was able to grow into the carbonized wood
[61]. A similar in vivo study was performed with bamboo charcoal. The results showed that charcoal bamboo as a bone substitute has good biocompatibility and osteoconductivity
[62]. Although pure carbonized wood had good biocompatibility and osteoconductivity, the complete loss of its mechanical properties made it an impractical material. Years later, the mechanical properties of pure carbonized wood were improved by an impregnation with silicon carbide (SiC) to produce a biomaterial called ecoceramics. In the preparation process, natural wood was pyrolyzed at 1000 °C using argon gas; the natural wood lost around 75% of its weight and 60% of its volume as a result of the treatment. The remaining scaffold was infiltrated with melted Si at 1550 °C. Si reacts with carbon in pyrolyzed wood to form SiC. Different wood species have been used to produce wood-based ecoceramics, for example, maple
[63], eucalyptus
[64], mango
[65], oak
[66], beech
[67], pine
[68], and others
[69]. The technique preserved the porous structure of the wood while adding the rigidity of SiC. It is also a light-weight material, with density around 1100–2300 kg/cm
3, depending on the selected wood
[65][66][67]. In addition, ecoceramics have great heat and electric resistance
[70][71]. Due to various properties, ecoceramics have attracted more interest of researchers in civil
[72], aeronautical
[73] and electronic
[74] engineering, and only a small number of studies consider ecoceramics as a material for medical applications. One in vivo study has been done with SiC scaffolds that were implanted in sheep metatarsal bones. Histological analysis was performed after 4, 8, 12, and 48 weeks. Analysis revealed good scaffold-to-bone adhesion, and new bone ingrowth inside the scaffolds was documented as well
[75]. Since then, few authors have proposed combining wooden scaffolds with other biomaterials. In one study, SiC scaffolds derived from beech, eucalyptus, and sapele were combined with bioactive glass. An in vitro study with MG-63 osteoblasts showed good cellular attachment to both coated and uncoated SiC scaffolds. Additionally, the osteoblasts proliferated equally in standardized environments and on the surface of bioactive-glass-coated SiC scaffolds
[76]. In another study, carbonized wood scaffolds derived from cane and pine
[77][78] or rattan
[79] were combined with hydroxyapatite (HA). The obtained samples showed the preserved porous structure and improved mechanical properties; the compressive strength reached 0.4 MPa and the tensile modulus increased 2–3 times
[77][78][79].
3.6. Cellulose-Based Scaffold
Few authors have considered wood as a base for cellulose-based scaffolds. To create such scaffolds, more extensive wood processing is required. Firstly, wood is processed into cellulose. Cellulose is a natural linear cell polysaccharide consisting of glucose (C
6H
10O
5)
n. (
Figure 3). It is the main component of cell wall in green plants and algae, and bacteria produce cellulose to form a biofilm as well. While the purest natural form of cellulose is cotton, where cellulose comprises about 90% of cotton’s mass, wood is made of around 57% cellulose and remains the main source for producing cellulose
[80]. As a natural raw material, cellulose has been used for fabrics and papers for hundreds of years, but only 185 years ago, in 1838, the chemical structure of cellulose was discovered and described by French chemist Anselme Payen
[81]. Since then, production of cellulose from wood stock is performed by chemically dissolving unwanted components such as lignin, short-chained polymers, etc. Cellulose is widely used for its porous structure and insolubility in water and organic substances in medical filters
[82], pharmacy
[83], and wound dressings
[84]. In the last few decades, cellulose has also attracted researchers’ attention as a potential biomaterial for medical applications, similar to using wood as an implant material. In the 1960s, implantation of cellulose sponges was used to study tissue inflammation and granulation formation shortly after implantation
[85]. Years later, in the 1990s, researchers began to investigate the long-term effects of cellulose implantation. Märtson, Viljanto et al. used industrial soft cellulose sponges derived from eucalyptus, birch, or oak. Cellulose sponges were tested in vivo in rat soft tissue, and histological examinations were performed consecutively after 1–60 weeks. Histological evaluation revealed that the inflammatory response of the surrounding tissues subsided after 4–6 weeks and revealed good connective tissue ingrowth into the cellulose sponges. The researchers also detected a slow resorption and degradation of the pure cellulose sponges
[86][87]. In addition, the biocompatibility of cellulose sponges with bone tissue was investigated in vivo; cellulose sponges were tested into the femoral bone cavity of rats. Bone ingrowth into cellulose sponges was recorded after 4–6 weeks
[88]. Later researchers started combining cellulose fibers with other biomaterials. An in vitro study was performed with chondrocytes from the bovine knee joint. Cellulose scaffolds were exposed to saturated calcium hydroxide (Ca(OH)
2) solution, then immersed in supersaturated simulated body fluid (SBF). Thus, a calcium phosphate coating was created. Although the cellulose and calcium phosphate scaffolds caused an acidic reaction in solution and the pH had to be adjusted with calcium hydroxide
[89], better cellular adhesion was detected compared to untreated cellulose scaffolds. In another study, cellulose fibers were impregnated with hydroxyapatite particles. Tomilla, Ekholm et al. published two studies on cellulose coating with hydroxyapatite derived from bioactive glass. Bioactive glass S53P4 (Abmin Technologies Ltd., Turku, Finland) was dissolved in SBF, and cellulose sponges were immersed in the SBF solution at 37 °C for 24 h. After 24 h of immersion in SBF, calcium hydroxyapatite was formed on the surface of the scaffold.
Figure 3. Cellulose molecule.