1. DHR via Turbocompounding
Turbocompounding can be viewed as the most mature technology to recover waste energy directly from exhaust gases. It tries to exploit the residual enthalpy owned by the exhausts, which generally leaves the engine at high temperature and also a not negligible pressure (1–4 bar
abs,
[1][2]), so making possible an expansion phase, usually realized by a dynamic turbine
[3]. Hence, it behaves as a turbocharger stage
[4], where the turbine is coupled to a compressor for engine intake air charging; alternatively, the recovery turbine can be mechanically linked directly to the engine crankshaft, giving power to the wheels
[5][6], or a further energy conversion with an electrical machine can be introduced exploiting the energy recovered in the electrical form
[7]. This configuration is interesting from a technical point-of-view since it consents to regulate the speed of the turbocharger in order to match the boost requirements of the engine, avoiding waste gate valves. It is particularly suitable for hybrid electric vehicles, where the optimization of a range extender engine can be maximized and the electrical energy recovered more easily stored and exploited
[8][9].
In order to increase the exploitation of the enthalpy of the exhaust gas, an electric motor-generator on the turbocharger shaft can be placed (
Figure 1a) building an easy direct recovery system. In this case, the increased complexity of the control system would correspond to the mechanical simplicity. In fact, the expansion should feed the compressor to reach the requested intake air boost pressure. The remaining power delivered by the turbine can be transformed into electrical power thanks to a motor/generator machine on the same shaft. During transient conditions, the electrical machine can boost the compressor, limiting the turbo-lag
[10][11]. The control system should have the boost pressure requested by the compressor as a controlled variable by estimating the mechanical power requested and leaving the residual mechanical power eventually delivered by the turbine to the electrical generator. The same control system, when the turbo-lag is present, will dispatch the mechanical power delivered by the electrical machine which behaves as an electric motor, accelerating the compressor speed. Critical aspects related to the reliability and efficiency of the electrical machines can be present, considering their high speed of rotation (100–150 kRPM) and the high operating temperatures that limit the capabilities of the electronics
[12].
Figure 1. Direct expansion recovery configurations: (a) electrical motor-generator on turbocharging; (b) parallel turbine and (c) series turbine.
A parallel turbine can be proposed (
Figure 1b), recovering also the enthalpy loss in the turbocharging control stage, conducted with a waste-gate or by varying the position of the inlet nozzle of the turbine (variable geometry turbine, VGT)
[13][14]. The management of the turbo-lag reduction is not possible being the auxiliary turbine not mechanically linked with the one which drives the compressor. Additionally, in this case, a control system shares the two exhaust gas flow rates, giving priority to the boost pressure. An additional turbine placed downstream of the turbocharger (
Figure 1c) seems the simplest possibility and it has the added value to be a kind of plug-in component, allowing a retrofitting system. The interference on the engine exhaust backpressure should not be neglected
[15].
Generally speaking, turbocompounded engines improve fuel economy with low weight requirements and low encumbrances on board. In every configuration presented the recovery is strictly dependent on the engine displacement and its working point considered, while the turbocharger regulation strategy (waste-gate or VGT) influences more the operating condition. The introduction of two-stages or twin turbines can improve the recovery and the fuel consumption reduction, but further increase the complexity of the layout
[16]. However, the final recovered power is demonstrated that could sum up to 18–20% of the engine’s brake power
[14][17][18].
Turbocompounding can also enable the engine to introduce delayed ignition timing, a higher EGR rate with reduced NOx, and faster time response during transients
[19]. The degree of freedom in engine design is increased by turbocompounding: several parameters can be optimized in the system, such as size, expansion ratio, efficiency and rotational speed of recovery turbine, exhaust manifold geometry, air-fuel equivalence ratio, engine boost pressure, engine compression ratio, intake and exhaust valves timings, the start of combustion timing, EGR systems, and exhaust after-treatment placement. In the development of turbocompounded engines and for the selection of the configuration, increased attention should be paid to the turbine efficiency and the augmented pumping work on the engine
[20]. In fact, when turbocompounding is considered, turbine efficiency plays a crucial role. Turbine design should consider variable geometry and revolution speeds to be set up to deal with various working conditions. The main limitations, indeed, are related to the low efficiency of the recovery turbine, which limits the useful power. The additional exhaust back-pressure on the engine represented by the crossing of the exhaust gas through the turbines leads to higher pumping loss seen by the engine which increases PMEP and reduces engine efficiency
[19][20] vanishing part of the recovery. The problem with the turbine efficiency is real since usually during engine operations its efficiency is sensibly low
[21]. Electrical machines also suffer low efficiencies when they rotate at high speed; hence, a gear or a Continuous Variable Transmission (CVT) could be used to reduce the shaft speed
[6][20] at the electrical machine side.
2. DHR via Inverted Brayton Cycle (IBC)
The inverted Brayton cycle is a novel recovery option not yet fully explored in the literature and with very few experimental tests
[22]. It makes use of the exhaust gases as a working fluid, so acting as a direct recovery. The gases are under-expanded below atmospheric pressure in a recovery turbine (sections 1–2 in
Figure 2). They are cooled down (sections 2–3 in
Figure 2) before the re-compression is required to bring them again at a pressure slightly higher than the environment to make their evacuation possible in the atmosphere (transformation 3–4 in
Figure 2). Hence, an IBC-based unit is composed of a recovery turbine, coupled to the same shaft with a compressor, and an IBC cooler between them (
Figure 2)
[23][24].
Tgas (or
T1) is the temperature of the gas considered for direct energy recovery (i.e., to be expanded): its value depends on the exhaust stream considered and the specific operating point. In order to be suitable for this kind of energy recovery, it should be higher than about 400 °C
[25], otherwise, the energy produced during the expansion is not sufficient to drive the IBC compressor and to have net positive energy recovered.
Tenv is the environmental temperature and represents the low boundary limit of the IBC:
T3 is the lower temperature of the gas reached within the IBC and it should be as close as possible to
Tenv, in order to reduce the power requested by the compressor as much as possible to bring the gas to environmental pressure. Therefore, the cooling phase from IBC sections 2 to 3 is crucial, but it is usually constrained by the thermal sink availability
[25]. Pressure after re-compression
p4 is close to the environmental one: only a slightly higher value (5–10 mbar) is needed to be sure of the evacuation of the gases. On the other hand,
p1 usually ranged from 1.2 to 2 bar
abs and depends on the specific layout of the engine exhausts (for instance, the presence of the turbocharging with its control device) and it is surely modified by the presence of the IBC turbine, which definitively imposes backpressure on the engine exhaust.
p3 is the subatmospheric value, and it cannot be pushed down to 0.3–0.4 bar
abs. Hence, from an energetic point of view, the low-pressure ratio in the turbine represents a drawback and limits the cycle efficiency and, finally, the overall IBC unit recovery efficiency, which is not higher than 8%
[26].
Figure 2. IBC layout and simple T-s diagram. Straight line is the IBC thermodynamic cycle, dotted one is the isobaric curve at environmental pressure, dashed-dot line is the isothermal line at atmospheriric temperature.
From a technological point of view, the efficiencies of both the turbine and compressor have a great influence on the final recovery, as well as the effectiveness of the IBC cooler
[25][27]. The need for a reliable control strategy on the rotational speed of the IBC turbine-compressor shaft is mandatory to guarantee gas evacuation to the environment, maximize efficiency and minimize the impact on engine backpressure and eventual turbocharging regulation
[28][29]. The vacuum management on board can represent an issue, but the possibility to exploit mature technology for machinery and other components makes this recovery option competitive with other opportunities with similar theoretical final recovered energy (in the range between 2 and 5% of the engine brake power)
[30][31]. Other concerns can be related to the presence of water condensation before final compression, but it can be managed quite easily considering the low pressure profiles
[32][33]. Moreover, the IBC does not prevent the possibility to introduce a cascade indirect recovery on the IBC cooler, combining two different recovery options
[34] or introducing a regenerative heat exchanger to improve the applicability to low temperature hot sources
[35][36]. Definitively, IBC applications are suitable for energy recovery in steady applications from internal combustion engines, such as industrial devices
[37][38], gas turbines and combined cycle power plants
[39][40], marine transportation, and CHP configurations
[41][42].
3. DHR via Thermoelectric Generation
The thermoelectric generator (TEG), as one of the WHR systems, can convert part of the wasted thermal power of the exhaust gas directly into electricity thanks to the Seebeck effect. So, it can be categorized as a direct recovery. Its main advantages are related to light-weight, silent operation, and compactness, making it more attractive for light-duty vehicles and passenger cars
[43], although applications on larger engines (marine transportation) have also been studied
[44]. This direct conversion is very interesting, but the very low efficiency of thermoelectric materials is the major problem, which leads to very low power recoverable
[45]. Despite the power producible from each TEG module being only a few Watts, a recovery efficiency equal to 7% of the exhaust gas energy has been demonstrated (so, similar values can be referred to the engine brake power), strongly depending on the material and its so-called figure of merit
[46][47][48].
The shape of the heat exchanger used for TEG can increase the backpressure on the engine exhaust, which raises the pumping mean effective pressure of the ICE, enhances the hot side temperature of the TEG and its maximum output power, and the effects on the brake power, brake torque, engine efficiency, and fuel consumption should be considered in an integrated way
[49]. The heat transfer on the cold side should also be improved, in order to increase the TEG efficiency: higher performance can be obtained by using enhanced cooling media, such as nanofluid-based ones
[50]. The thermoelectric cells could be arranged according to different shapes in order to make different contributions possible from different exhausted heats. An easy recovery, for instance, could be realized opportunely by shaping the heat exchanger, substituting the ones present on the cooling or lubricating circuits, and also on the exhaust gas
[51][52].
Its application in hybrid vehicles is also interesting. In an urban driving cycle, the application of TEG in a series of hybrid vehicles shows better fuel economy than with integration into the conventional vehicle. However, in a highway driving cycle, the fuel-saving effect of the thermoelectric generator integrated into the conventional vehicle is better
[53]. The combination with other energy recovery in cascade opportunities is possible since a certain amount of thermal energy is still available
[54].
4. IHR via Thermodynamic Cycles
As already presented, when the energy recovery is realized with a different fluid, and not acting directly with the exhaust gases, it can be categorized as indirect heat recovery. This principally referred to recovery options that use the thermal power of the exhausts to feed a bottomed thermodynamic cycle whose net output is the production of work and residual heat to be discharged at low temperatures. On the gas side, the heat exchanger used to capture the exhaust energy is the crucial component. It should be small, and light, with high exchange coefficients, low thermal inertia, and very low pressure drops
[55][56]. This latter is the main limitation, since the pressure drop produced on the exhaust side causes a backpressure increase on the engine, with higher fuel consumption as a consequence
[57][58]. The reduced weight avoids the increase in the propulsion power of a vehicle and a suitable design can reduce the incumbrance which is a problem on vehicles of reduced dimensions. Definitively, hot and cold sources are represented by exhaust gases and the environment, so requiring optimized heat exchangers.
Few cycles can be used for this purpose: Rankine cycles are the most studied, in particular with organic fluid as working fluid (ORC) due to the low boiling temperature which corresponds to low pressure values which makes easier and cheaper the construction of the recovery unit. Additionally, the Brayton cycle can be used when the thermodynamic working fluid is a gas, but it is not so studied in the transportation sector. Other innovative cycles try to improve the overall efficiency of the recovery, introducing cascade cycles, internal heat exchangers and multiple evaporation pressures, zeotropic mixtures as working fluids and wet expansion in Trilateral flash cycles (TFC). The Stirling cycle has also been proposed for its quite simple implementation.
4.1. Organic Rankine Cycles
The Organic Rankine Cycle (ORC) is by far the most studied opportunity in waste heat recovery: it is very similar to the steam Rankine cycle but uses a working fluid of an organic nature (Figure 3a,b). This consents to the recovery from low-grade thermal sources [59]. An ORC-based recovery unit is composed of a heat exchanger acting as an evaporator (or Heat Recovery Vapor Generator, HRVG, from section 2 to 3), an expander or turbine (state points 3–4), a condenser (4–1) and a pump (1–2). Some other components can be introduced for efficiency optimization or plant operability (such as a tank/reservoir, a bypass branch for the turbine, an internal heat exchanger for the regeneration stage, etc.) [60].
It is quite commercial for different applications (industrial, geothermal) but it has been studied only in recent years for the transportation sector, in particular for heavy-duty applications. For light-duty and passenger cars, the higher variability of the waste heat temperature and amount (for instance, exhaust mass flow rate) makes it difficult to implement a high-efficiency recovery plant in operating conditions [61].
Figure 3. (a) base T-s diagram of an Organic Rankine Cycle bottomed to exhaust gas and using R245fa as working fluid (dashed line is the saturation curve of the fluid)); (b) base layout of ORC unit.
4.2. Trilateral Flash cycle (TFC)
Trilateral Flash Cycle (TFC), or Organic Flash Cycle (OFC), is a bottoming thermodynamic cycle particularly suitable for low-temperature waste heat recovery, around 80–100 °C [62][63]. The name “Trilateral” highlights the shape of the cycle, which is almost triangular (Figure 4) and it is particularly suitable for that kind of upper thermal sources that do not match with a Rankine or Hirn cycle. So, it can be interesting for very low temperature sources and limited flows of fluid cooling, or for cascade cycle, bottoming to a main recovery unit to further increase the final energy produced. The shape of the T-s diagram at the saturated liquid side can add some additional interest to the exploitation of this technology. It is based on a Rankine cycle in which the vaporization is not realized, but a saturated liquid is expanded. Hence, it is composed of a pump, a simple heat exchanger used to heat up the high pressure working fluid to the saturated liquid state and so, a wet expansion within the two-phase region and a final condensation [64].
The difficulties to recover energy in the two-phase region of the fluid (wet expansion) suggest using volumetric machines as expanders and, preferably, with a variable volume ratio [65]. As shown in Figure 4, the possibility to increase the exergy recovered is great (i.e., the closer the value of the TFC to the upper and lower thermal source/sink), but only if the expander efficiency is close to the one of a conventional turbine; TFC can compete with ORC in terms of final energetic efficiency [66]. More recently, the use of fluid mixtures has also been proposed to increase the efficiency of the OFC [67].
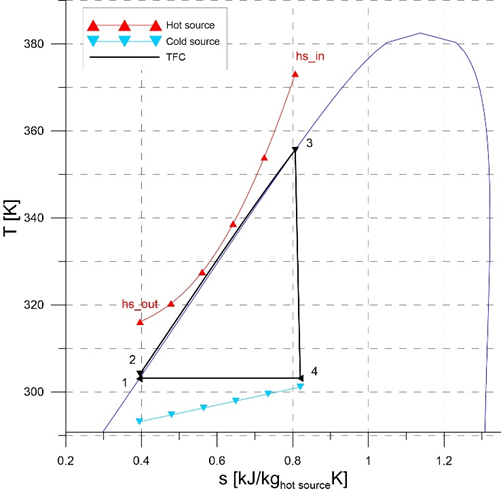
Figure 4. TFC T-S diagram [68]: transformation 1–2 is a pressurization in the liquid phase, 2–3 heating of the liquid, 3–4 is the wet expansion, 4–1 the condensation. Dark blue line is the saturation curve of the working fluid (R1234ze(E)).
4.3. Use of Zeotropic Mixtures as Working Fluids
The selection of working fluids is one of the most investigated issues. It should respect several constraints and parameters: low flammability, no toxicity, low ozone depletion potential and global warming potentials, as well as other low environmental impacts. At the same time, the working fluid should have high thermodynamic performances in relation to the upper and the lower thermal sources available. Dry and isentropic fluids are suitable for ORC-based units [69]. The opportunity to be mixed with lubricating oil is an additional positive issue because volumetric expanders which are very suitable for small-size recovery units need to be lubricated to improve mechanical and volumetric efficiencies.
In this regard, the opportunity to use zeotropic mixtures (i.e., mixtures of fluids, which can vary their temperature during phase change) can have the opportunity to better approach the thermal sources and sinks during evaporation and condensation of the working fluid, thanks to the temperature glide during phase transition (Figure 5) [70].

Figure 5: T-s diagram of an ORC with mixtures of working fluids [71]. Inset zoom on the temperature glide in the evaporation phase. Similar to the condensation one. Dashed line is the saturation curve of the fluid mixture (R245fa/benzene 0.95/0.05)
4.4. Cascade Cycles
When the temperature of the upper thermal source is enough high (>300 °C, for instance), only ORC is not suitable, since it introduces a high exergy destruction rate in the heat recovery vapor generator [72]. Indeed, the influence of the pinch point temperature on the energy recovered is significant and the differentiation of the layout can be proposed to increase the recovery efficiency [73]. In particular, the combination of more than one thermodynamic cycle can be used, in a cascade form [74], in order to match the upper thermal level with one recovery section, and the cold sink with a bottomed one, increasing the exergy efficiency of the overall recovery unit [75].
The use of the CO2 Brayton cycle is very popular, where the CO2 is performed in the supercritical phase (pc,CO2 = 74 bar). In particular, the CO2 is in a dense phase when it is close to the critical point, with a thermodynamic advantage in terms of fluid density (it has a density close to the one of a liquid and it has low viscosity like a gas, increasing the mass per unit volume without increasing pressure drops across ducts) and reduced viscosity with respect to a gas. Moreover, CO2 is a natural compound, stable, non-toxic and non-flammable, with a reduced environmental impact with respect to fluorinated gases. Thus, the supercritical CO2 (sCO2) topping cycle is bottomed by an ORC one, aimed to partially recover the thermal energy to be disposed of in the lower CO2 pressure side (Figure 6a, [75]), and a trade-off between the cycle efficiency and the heat recovery must be analyzed, [76]. Only a few sCO2 recovery plants have been manufactured and actually operated since this technology has not yet reached technical and commercial maturity [77]. Several configurations have been proposed in this regard (parallel, cascade [78], regenerative [79][80], re-heated and intercooled [81][82], recompression [83], dual expansion [84][85], etc.) in order to increase the net power output. The complexity of the recovery plant is shown in Figure 6b, where the two cascade sections are sketched. State points from 1 to 5 refer to sCO2 Brayton cycle, while 7 to 9 are referred to ORC one. The need for an additional heat exchanger (CO2 cooler after HRVG, from state points 5 to 1) is highlighted. The management of supercritical values of CO2 pressures can also represent a limiting factor, in particular for small-scale units, where the amount of recovery does not justify high-pressure components, piping, seals, etc., which in turn brings higher costs. In fact, the introduction of ORC as the bottoming cycle in combined recovery plants has been proposed also for medium-low temperature sources, to increase the overall energy recovered [86].
Figure 6. (a) example of T-s diagram of a combined energy recovery unit composed by supercritical CO2 and ORC [75]; (b) simple layout of sCO2+ ORC unit.
4.5. Stirling Cycle
Even though the Stirling engine was discovered in the early 1800s, only recently it has been applied in several applications, especially in combination with renewable sources and as a waste heat recovery option in industry [87][88]. The Stirling cycle is a gas engine cycle composed of two isotherms and two isochore lines as an indicator diagram. A regenerator is interposed in between. Its main advantage is that the Stirling cycle does not replace the working fluid for every cycle. The working gas can be air or other gases. The overall amount of thermal energy is supplied externally from the engine, making possible the use of any kind of source. Stirling engines can achieve high thermal efficiencies, ideally the one of the Carnot cycle, since the heat exchange takes place at a constant temperature. In reality, the transformations are usually far from the ideal ones, and the real efficiency does not overcome 20–25% during operation [89].
Stirling engines could be an interesting solution for ICE thanks to their compact design, easiness of management, application flexibility and the possibility to be adopted for different energy sources [90][91]. The power recoverable can range from hundreds of watts to kW. Different solutions of Stirling engines can be found, such as alpha, beta, and gamma configurations [92], single or double-acting cylinders [93], or free-piston operational mechanism [94].
5. Direct Heat Utilization
The thermal power recovered can be directly used for heating purposes, in particular for steady state applications in CHP mode. The first example usually considered, is cabin heating in automotive applications [95]: the heat removed from the engine by the coolant is partially used in a heat exchanger placed in the dashboard of the vehicle. This exchanger is crossed on one side by the hot coolant, exiting from the engine jackets, and by air on the second side, thanks to the controlled fan, which regulates the thermal power to be sent to the cabin interior. Often, the cabin heater lays in a branch of the cooling circuit in parallel with the radiator and the bypass branches, without undergoing thermostat control [96]. Additionally exhaust heat or lubricating heat can be used for this purpose, in order to optimize the thermal level [97][98]. Its integration with other thermal needs improves the overall efficiency of the system [99], for instance, accelerating the warming up of the lubricant oil, reducing frictions, and improving engine thermal management: the use of the exhaust to warm up the engine oil during cold phase demonstrated a fuel consumption reduction over 3% [100][101].
A different use of the thermal power to be disposed on board is represented by absorption chillers, which can improve the integration concept of thermal needs [102][103], feeding refrigeration, and cooling needs. Waste heat driven heat pumps can also allow low-temperature heating purposes [104][105]. The integration of absorption chillers on board can also be used for increasing the propulsion system efficiency, cooling the engine charge air [106], or optimizing the thermal management of electric devices [107][108]. In industrial applications, the integration of Rankine cycles with multiple-level refrigeration systems can meet the requirements of air-conditioning, refrigeration, and also cryogenic cooling, aiming at a full energy recovery of lost heat [109].
When applied to exhaust gases, the heat exchanger performance plays a crucial role: it should have high thermal efficiency to maximize the thermal exchange towards the working fluid of the recovery unit [110], and also in terms of backpressure increase on the engine, which should be significantly limited in order to avoid excessive overconsumption on the engine itself and tailpipe emissions [111]. The impacts of extra weight, additional cooling fan power consumption, transient control, effects on engine intake air management as well as exhaust after-treatment thermal inertia should be also considered in mobile applications [112].