The Bacillus subtilis (B. subtilis) group is a ubiquitous Gram-positive bacteria with a remarkable adaptability potential that enables it to survive in highly diverse environments.
1. Introduction
The B. subtilis group consists of four species, including Bacillus subtilis, Bacillus pumilus, Bacillus licheniformis, and Bacillus amyloliquefaciens, discovered several decades ago. Over time, numerous new species and subspecies have been described based on molecular evolution, physiology, and chemotaxonomic characterization [1]. B. subtilis is a model organism used to investigate cell motility, biofilm formation, protein secretion, cell division, secondary metabolites biosynthesis, adherence to plant root and fungal hyphae, cytoplasm altercation via intracellular nanotubes, and kin-recognition [2]. In biotechnological industries, B. subtilis is a popular workhorse used for the biosynthesis of a broad range natural products, from enzymes to purified bioactive compounds [3][4]. Its natural competence to genetic engineering and well-described gene expression system makes it attractive on many occasions [4]. Moreover, recently, it also earned attention as a biocontrol agent in agronomy by antagonizing phytopathogens and promoting plant growth [5].
The impressive skill set of B. subtilis for producing diverse bioactive metabolites was recognized in the last decade. It has been demonstrated that ~5% of a wild-type B. subtilis genome is exclusively devoted to the synthesis of bioactive compounds [6]. For a long time, it was considered for the production only of cyclic peptides such as iturins, surfactins, and fengycins [7][8]. Nonetheless, due to the discovery of numerous antimicrobial exhibiting linear lipopeptides, PKs, and volatile metabolites, it has gained a high commercial interest. The versatile bioactive metabolites produced by the B. subtilis group may be classified based on several criteria, including their biosynthetic pathways, function, structure, source, physicochemical properties, molecular targets, or bonding patterns [9].
2. Non-Ribosomal Peptides (NRPs)
NRPs are molecular assembly machines that use multi-modular enzyme complexes rather than a DNA template to construct a protein
[10]. A module is a part of the non-ribosomal peptide synthetases (NRPS) enzymes that assimilates the amino acid into a peptide backbone of a specific kind. Further, each module may be divided into three domains, adenylation (A), thiolation (T) or peptidyl carrier protein (PCP), as well as a condensation (C) domain that catalyzes the individual steps of NRP synthesis
[11] (
Figure 1).
Figure 1. Biosynthesis of non-ribosomal peptides (NRPs), consisting of core and auxiliary domain. Core domain encoding NRP includes A; Adenylation, PCP; peptidyl carrier domain, C; condensation ans TE; thioesterase domain. Auxiliary or optional domains responsible for cyclization (Cy), N-methylation (MT) and epimerization (E).
The NRPs may be synthesized via a multi-enzyme thio-template mechanism or without the multi-enzyme
[12]. The NRPs synthesized through a thio-template are usually 2–50 amino acids and some other moieties such as a fatty acid chain, whereas the NRPs synthesized without a multi-enzyme template are comparatively smaller
[13]. Based on biosynthetic pathways, NRPs can be divided into thio-template and non-thiotemplate NRPs.
2.1. Thio-Template NRPs
The thio-template NRPs can be classified as cyclic lipopeptides and non-cyclic or linear lipopeptides based on their chemical structures.
2.1.1. Cyclic Lipopeptides
Thio-template non-ribosomal cyclic lipopeptides were first isolated during the 1950s–1960s from
Bacillus spp. The cyclic lipopeptides are primarily synthesized via the sequential addition of residues, either in an iterative or a non-iterative manner. The lipopeptide synthesis pathways are very flexible; therefore, the synthesized peptides are incredibly diverse in nature. The cyclic lipopeptides produced by
B. subtilis can be classified into four classes
[14].
-
Surfactins: Surfactin was first isolated in 1968 from the culture supernatant of
B. subtilis, which exhibited an excellent biosurfactant activity
[15]. Subsequently, surfactin was demonstrated to be an antitumor, antibacterial, anticoagulant, and hypocholesterolemic agent
[16] as shown in
Figure 2.
-
Iturins: In 1949, Walton and Woodruff isolated the first antifungal iturin from
B. subtilis. Later on, in 1950, a second similar compound iturin was reported, whose name was derived from Ituri (the name of the place in Congo where the soil sample was collected)
[17]. The exact structure of iturin was elucidated to be a cyclic hepatolipopeptide attached to the alkyl chain (
Figure S1). Iturins are known to display potent antifungal activity and could be used as an active ingredient in several phytopathogen control products. The closely related cyclic lipopeptides could be classified as iturin: bacillomycin L
[18], mycosubtilin
[19], bacillomycin D, bacillomycin F
[20], mojavensin A
[21], and subtulene A
[22].
-
Fengycins: In 1986, Japanese and German scientists simultaneously discovered fengycin from
B. subtilis [23]. Initially, it was determined that fengycin inhibits the growth of filamentous fungi and is ineffective against non-filamentous fungi and bacteria. Later on, however, its antiviral
[8], antibacterial
[24], and anticancer properties were reported
[25]. It also exhibited a plant growth-promoting property, which is desirable in the agriculture industry.
-
Kurstakins: Kurstakin is a lipo-heptapeptide exhibiting antifungal activity produced by several
B. subtilis strains. Kurstakins cannot be recovered from the culture supernatant but are found in association with the producing cells
[26]. Nevertheless, the co-infection study conducted with the producing and non-producing strains demonstrated that it is extracellular
[27]. This contradiction suggests that kurstakin is an extracellular metabolite having a high affinity to the cell membrane. This affinity is probably due to the presence of histidine, which gives a positive charge to kurstakin and allows its electrostatic interaction with the phospholipid of a membrane.
-
Plipastatins: Plipastatin was first reported from
B. cereus as an antiphospholipase, before being identified in
B. subtilis [28]. Plipastatins are closely related to fengycin. The alteration happens from the inversion of two stereocenters, offering a distinct 3D structure to plipastatin’s backbone. Notably, these apparent small structural differences result in a loss of antifungal activity
[29].
Figure 2. Potential application of secondary metabolites synthesized by Bacillus subtilis.
2.1.2. Linear Lipopeptides
Gageopeptides
Recently, several linear lipopeptides have been reported and characterized from B. Subtilis. For instance, Gageostatin was reported from a marine-derived B. subtilis [30]. Gageostatin consists of 3-beta hydroxyl fatty acid attached to heptapeptide. It is composed of the same residues as reported for surfactin. However, differences were found in their structures and molecular masses. Gageostatins were found in linear form with exclusively L-leucine, while surfactins are cyclic lipopeptides with L and D-leucine.
Siderophores
Siderophores are small molecules having a high affinity toward ferric iron. Besides iron scavenging, they are also used to form stable complexes with environmentally important metals. Based on their chemical moiety, siderophore can be categorized into three types, i.e., hydroxamate, catecholate, and carboxylate siderophores. Most of the siderophores produced by bacteria are catecholate, and few are carboxylate and hydroxamate [31]. Bacillibactin is a well-known catecholate siderophore produced by different B. subtilis strains that exhibits strong antibacterial properties and moderate cytotoxicity [32]. Bacillus spp. are widely studied for the synthesis of bioactive metabolites. However, their siderophore-producing capabilities have not yet been much explored. Previously, a siderophore-producing Bacillus spp. was reported that enhanced the bioremediation of metals and increased plant growth. B. subtilis strain CAS15 was isolated from the rhizosphere and identified as producing siderophore, and it also inhibits the growth of phytopathogens [33].
2.2. Non-Thio-Template NRPs
B. subtilis is capable of synthesizing antimicrobial NRPs via non-thio-template mechanism. Rhizocticins are non-thio-template peptides that consist of an arginine linked with L-2-amino-5-phosphono-3-
cis-pentanoic acid. Sometimes they are supplemented with leucine, isoleucine, and valine
[34]. Besides rhizocticins,
B. subtilis can also synthesize dipeptide NRPs such as bacilysin (tetain) and chlorotetain. Regardless of their simple composition (L-alanine link with L-anticapsine), they exhibit strong antibacterial and antifungal activity
[35]. The antibacterial activity is mediated by the L-anticapsine, which inhibits the synthesis of glucosamine-6 phosphate. Its inhibition stops the synthesis of peptidoglycan, which is a major constituent of the bacterial cell wall. For antifungal activity, it has been suggested that anticapsine can suppress the biosynthesis of chitin and fungal cell membrane mannoproteins
[36]. Tetain and chlorotetain are shown to inhibit the growth of
Aspergillus fumigatus and
Candida albicans [37]. Mycobacillin and bacitracin are non-thio-template polypeptides produced by
B. subtilis.
3. Ribosomal Peptides (RPs)
Ribosomal peptides (RPs), also known as ribosomally synthesized and post-translationally modified peptides (RiPPs), are derived from a relatively short precursor peptide and are matured through post-translational modification
[38]. Several enzymes are involved in these modifications; thus, structurally diverse peptides are generated. In order to classify the RiPPs produced from
B. subtilis, several classifications have been proposed, and the classification based on the biosynthetic pathway or chemical structure is reasonable, as reported for bacteriocin produced by
Enterococcus spp. and
Streptococcus spp.
[39]. Consequently, the known
B. subtilis producing RiPPs could be classified into three main classes and several subclasses.
3.1. Class I—RiPPs
Class I consists of biologically active short peptides that are ribosomally synthesized and undergo post-translation modification (PTM), resulting in a unique structure and properties. According to PTM differences, class I can be divided into several subclasses.
3.1.1. Lanthipeptides
Class 1 lanthipeptides consist of highly diverse post-translationally modified peptides that characteristically contain cross-link thioether between non-proteinogenic lanthionine and 3-methyllanthionine
[40]. Lanthipeptides are produced as precursor peptides and consist of a leader and a core peptide. The precursor peptide is post-translationally modified and cross-linked with thioether, and, subsequently, the leader peptide is removed, and a mature lanthipeptide is released
[41]. Lanthipeptides exhibit promising antimicrobial activity, and, indeed, a lanthipeptide “nisin” is commercially used in the food industries. Nisin prevents the synthesis of peptidoglycan transglycosylation and forms a membrane-spanning pore
[42].
3.1.2. Lasso Peptides
Lasso peptides are a relatively newly characterized class of RiPPs composed of short-chain peptides comprising an N-terminal macrolactam by which the C-terminal is linked
[43]. The N-terminal “ring” is formed by 7 to 9 amino acids that are linked by an isopeptide bond between the N-terminal amino group of the first residue and the carboxylate chain of glutamate or aspartate residue
[41]. The first residue of lasso peptides, cysteine or glycine, is highly conserved. Therefore, bioinformatics approaches are recommended for the discovery of new lasso peptides
[44]. However, few lasso peptides have been discovered with alanine or serine as their first amino acid
[45].
3.1.3. Sactipeptides
Sectippetides are biologically active peptides with exceptional cross-links between the sulfur of cysteine and the alpha carbon of other residues catalyzed by S-adenosylmethionine (SAM)
[46]. The post-translational link of a thiol group to the alpha carbon is unusual in RiPPs and is responsible for the antimicrobial activity of sactipeptides
[47][48]. Genome mining can efficiently detect the unique SAM enzyme whose coding genes are co-localized with the sactipeptides biosynthesis gene cluster
[49].
3.1.4. Linear Azole-Containing Peptides (LAPs)
The linear azole-containing peptides (LAPs) are heterocyclic peptides derived from the threonine, cysteine, and serine of a short precursor peptide
[50]. They consist of four obligatory modules: a precursor peptide that is also known as peptide A, a heterotrimeric enzyme complex (dehydrogenase B), and cyclodehydratase C and D. Plantazolicin is a LAP produced by
B. amyloliquefaciens. Its biosynthesis initiates with the formation of azoline heterocycles via the C and D complex from threonine/serine and cysteine, followed by dehydrogenation by B leading to the synthesis of the corresponding azole
[51][52]. Regardless of the low amino acid identity of the BCD complex between LAP loci, numerous reports revealed that BCD genes could be associated with various LAP biosynthesis pathways by converting the precursor peptide into the active RiPP
[52][53].
3.1.5. Thiopeptides
Thiopeptides are an emerging group of antibiotics and include more than 100 compounds. Thiopeptides not only exhibit antibacterial activity but also possess broad bioactivities such as anticancer, antiplasmodial, and anti-immunosuppressive activities
[54].
3.1.6. Cyclic (Head-to-Tail) Peptides
The head-to-tail cyclic peptides are relatively long peptides with 35–70 amino acid residues. The peculiar features of cyclic peptides are not only their large size but also the modifying enzymes associated with their cyclization. Due to their macrocyclization, these peptides are relatively resistant to higher temperatures, pH changes, and several proteases
[55]. These peptides are distinguished from lanthipeptides in that they do not contain lanthionine, methyl lanthionine, and hydrated amino acid residues
[56].
3.2. Class II Peptides
3.2.1. Pediocin-like Peptides
The pediocin-like peptides inhibit numerous clinically relevant pathogens and have a conserved YGNGVXC motif. Despite their great potential as antibacterial agents, the problems associated with their commercial-scale production limit their industrial application. So far, coagulin is the single complete characterized pediocine-like peptide reported from
B. coagulans [57].
3.2.2. Other Non-Modified Peptides
The non-modified peptides have a conserved motif of DWTXWSXL at the N-terminus. In 2001, a hydrophobic and thermotolerant antimicrobial peptide, lichenin, was purified and characterized from
B. licheniformis culture
[58]. Recently, the biosynthetic gene clusters encoding the class IIb non-modified peptide BhIA were reported from the
B. subtilis,
B. lechniformis,
B. pumilus, and
B. amyloliquefaciens genomes via genome mining approaches. The structural analysis revealed significant similarities with holins produced by
Geobacillus spp
[59]. Holins are phage-encoded peptides involved in the disruption of bacterial cell membranes
[60]. However, the function of each biosynthetic coding module remains unknown. The holin-like BhIA metabolite exhibited antibacterial activity against pathogenic Gram-positive bacteria such as
Micrococcus luteus and multi-drug resistant
S. aureus [61]. The peptide BhIA is composed of 70 amino acids with a transmembrane domain at the N-terminus. Several hydrophilic amino acid residues at the N-terminal and specific membrane topology distinguish BhIA from holin
[61].
3.2.3. Large Antimicrobial Peptides
The large antimicrobial peptides are relatively larger and include bioactive metabolites having a size of more than 10 kDa. Their biosynthetic gene cluster consists of an immunity gene and a structural gene. Several
Bacillus species, such as
B. thuringiensis,
B. coagulans, and
B. cereus, are reported to produce large antimicrobial peptides
[59]. However, as yet, none have been reported from the
B. subtilis group.
4. Polyketides (PKs)
Polyketides are structurally diverse bioactive metabolites that contain an alternative methylene and carbonyl group
[62]. Polyketides are widely used as therapeutic agents to treat numerous diseases
[63]. For instance, tetracycline and erythromycin are used as antibacterials
[64], amphotericin is used as an antifungal
[65], and anthracyclin is used as an antitumor drug
[66]. The biosynthesis of PKs is carried out by a multi-domain enzyme, which consists of ketosynthase, acyltransferase, and thioesterase. Its biosynthesis is initiated by loading the acyl CoA on acyl carrier protein (ACP), catalyzed by acyltransferase (AT). The ketosynthase (KS) extends the carbon chain via decarboxylative condensation. The ketoreductase (KR), enoyl reductase (ER), and dehydratase (DH) domain may further modify the beta-keto group to produce diverse PKs (
Figure 3).
Figure 3. Schematic representation of enzyme domains involved in the biosynthesis of Polyketide (PKs). Core and auxillary (optional) domains are color coded. Core domains: AT; Acyltransferse, ACP; Acyle carrier protein, KS; Ketosynthase and TE; Thioesterase. Auxiliary domains: KR; Ketoreductase, DH; dehydrase, and ER; Enoyl acyle reductase.
4.1. Polyenes
Difficidins
Difficidins are unsaturated macrocyclic polyene synthesized by the type 1 PKS. Oxydifficidin is an oxidative form of difficidin having an additional hydroxyl group at position 5 [67]. It is encoded by the diff operon that has 14 open reading frames. Several KR, DH, and ER domains are missing within the diff operon and deviate from the colinearity rule. Moreover, the function of diffJ and diffK are unknown, and their activities do not appear in the final product [68]. Difficidin has broad-spectrum antibacterial activity and inhibits the biosynthesis of protein in E. coli [67][69].
Difficidins
The antibacterial exhibiting polyketide aurantinin A and B were isolated initially from Bacillus aurantinus
[70]. Recently, along with these two aurantinins, C and D were reisolated in combination with the genome mining approach
[71]. The structure of aurantinin and its analogues are very unusual, as they have 5, 6, 7, and 8-membered rings with a highly diverse tail. Nevertheless, the absolute structure is yet not elucidated, leaving numerous questions about conformation unanswered. Aurantinin B, C and D exhibit promising antibacterial activity against
Clostridium sporogenes and
S. aureus. However, they did not show any cytotoxicity against human epithelial colorectal adenocarcinoma and cellosaurus cell lines. The antibacterial mechanism was examined and revealed that aurantinin B-D disrupts bacterial cell membranes causes breakage of cytoplasm and leads to cell death
[71].
Macrolactins
Macrolactins and their derivatives (7-
O-succinyl or 7-
O-malonyl) are synthesized via a type 1 PKS. Macrolactins inhibit the growth of bacteria and have been isolated from various species such as
Bacillus sp. strain AH1591-1 and
B. amyloliquefeciens strain FBZ42
[72][73]. Macrolactins usually consist of 24 lactone rings and three diene moieties in the carbon skeleton. Its biosynthetic gene cluster mln composed of nine operons and 11 KS domains with acetate and malonate is the only used building block. Like
dif gene cluster organization,
mln appears in an occasional splitting of the modules. The second module is split between
mlnB and
mlnC, and a similar arrangement can be observed for modules number 5, 7, 8, and 10. A comparison of catalytic domain organization revealed that the ER domain is missing in module number 2, while two DH domains are missing in modules 7 and 10
[72].
4.2. Enediynes
To date, the PK enediyne is the most cytotoxic natural product, and its application as an anticancer drug has been clinically demonstrated
[74]. Due to the substantial cytotoxicity of enediyne, its application is minimal. However, its application in various antibody-drug conjugates and polymer-based drug delivery systems has had great success
[75][76].
5. Hybrid Metabolites
Hybrid metabolites are the products of biosynthetic pathways that comprise both (NRPS/PKS) types of modular enzymes. Questions relating to the synthesis of hybrid products are of great present-day interest, as their answer concerns genetic engineering efforts. Both depend on thio-template for acyl chain elongation and monomers triggering.
Macrolactins
Bacillaene has a linear structure and was first reported from
B. subtilis strains 55,422 and 3610
[77]. It is encoded by a hybrid PKS-NRPS biosynthetic gene cluster known as bacillaene PksX synthase (
Figure 4). The
pksX mega gene cluster in
B. subtilis 168 genome consisted of 5 open reading frames named
pksJ,
pksL,
pksM,
pksN, and
pksR [68][78]. The first two adenylation domains of
pksJ incorporate glycin and α-hydroxy-isocaproic acid. The third adenylation domain (
pksN) is responsible for the incorporation of alanine.
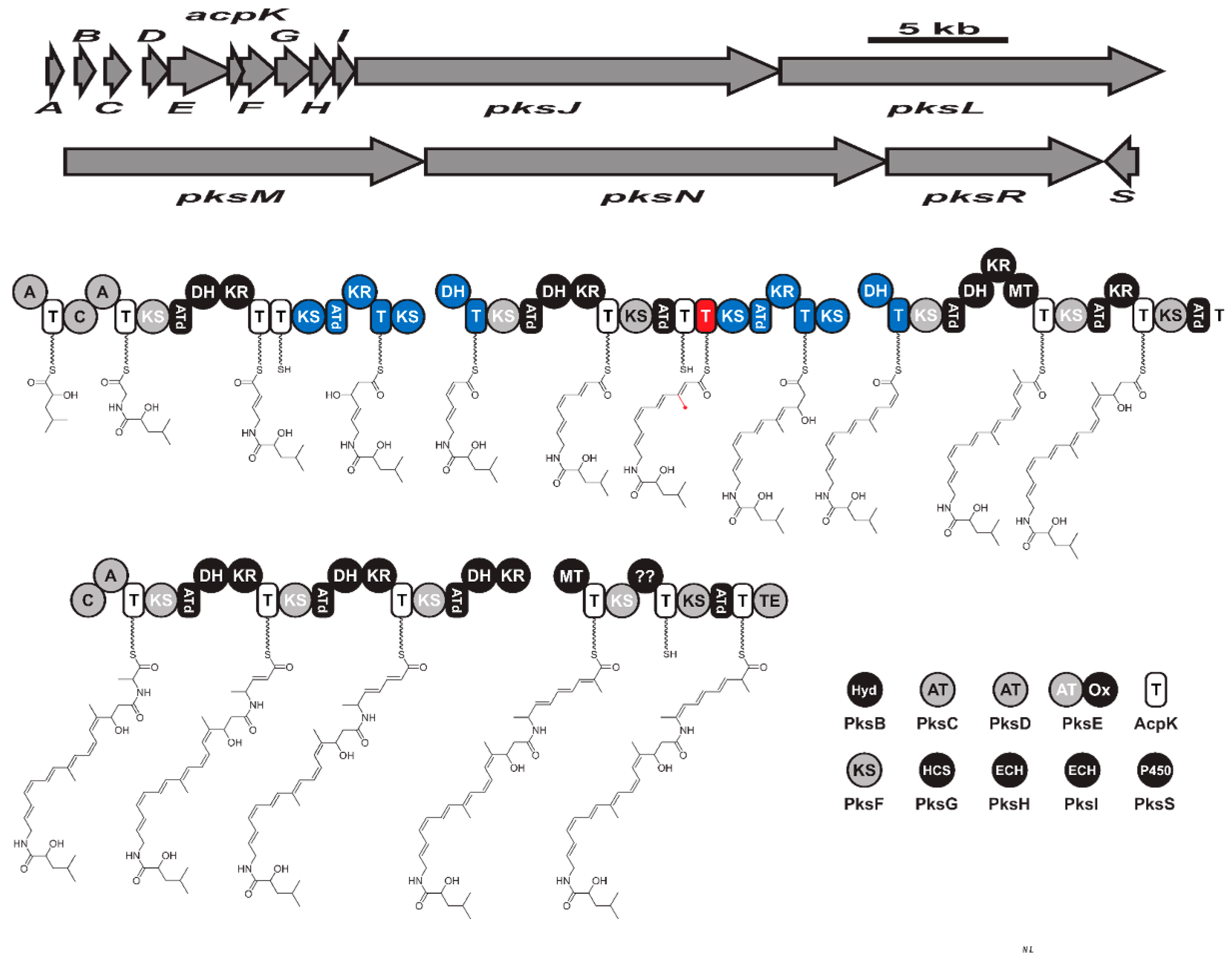
Figure 4. The biosynthetic gene cluster (pksX) and biosynthesis of hybrid bacillaene. Key: KS (ketosynthase), AT (acyltransferase), T (thiolation), DH (dehydratase), KR (ketoreductase), MT (methyltransferase), A (adenylation), C (condensation), ATd (AT-docking), Hyd (Zn-dependent hydrolase), Ox (flavin mononucleotide-dependent oxidase), HCF (HMG-CoA synthase), ECH (enoyl-CoA hydratase), and TE: (thioesterase).
Isocoumarins
Isocoumarins form a large, diverse class of biologically active metabolites with more than 200 metabolites
[79]. However, fewer are reported from
B. subtilis. It has been reported that
B. subtilis specifically produce isopropyl-8-hydroxy-3,4-dihydroisocoumarins with an active side chain or functionalized amino acid
[80]. Amicoumacin A-C (
Supplementary Material Figure S3E,F) and amicoumacin F are dihydroisocoumarins initially reported from
B. pumilus [81][82][83]. Later, they were reported from
B. subtilis and determined its strong antibacterial activity against the gastric pathogen
Helicobacter pylori [84]. Biosynthesis of these bioactive metabolites has been recently reported from
B. subtilis 1779 via a genome mining approach
[85]. The BGC encoding amicoumacin was predicted to be 47.4 kb in size and consists of 12 open reading frames. Further, the investigator expressed the biosynthetic gene cluster in a heterologous host and obtained amicoumacin A–C. Based on these findings, they predicted the biosynthetic pathway for amicoumacin to be synthesized via hybrid PKS-NRPS modular enzymes
[85]. The biosynthetic gene cluster consists of eight modules that synthesize two discrete pre-amicoumcin molecules. The unique dihydroisocoumarins core is likely to be synthesized via removing the AmiJ-M megasynthase complex to produce an oxygenated PK chain that reorganizes into a cyclic dihydroisocoumarin
[85]. Amicoumacin exhibit promising anti-inflammatory and antibacterial activity and has gained increased interest as a pro-drug candidate. Specifically, amicoumacin A is more attractive due to its potent anticancer activity and antibacterial activity against MRSA, with MIC less than 1 µg/mL
[82][83]. The antibacterial mechanism of amicoumacin was recently shown to be bind on the ribosome and inhibit protein biosynthesis
[86][87]. Amicoumacin A and C exhibit antibacterial activity, while amicoumacin B is non-antibacterial. Interestingly, the hydroxy group in amicoumacin A and C is responsible for their antibacterial activity as the phosphate ester-containing amicoumacin B lacks antibacterial activity
[88].
6. Volatile Metabolites
6.1. Volatile Inorganic Metabolites
The volatile inorganic metabolites are a by-product of the primary metabolites. They are usually nitrogen and sulfur-containing compounds such as ammonia (NH
3), hydrogen sulfide (H
2S), and hydrogen cyanide (HCN). Nitrogen-containing compounds are mainly emitted in the top sediment layer by denitrifying
B. subtilis strains. The
B. subtilis group also produces nitric oxide, which induces a systemic acquired resistance in plants against
Ralstonia solanacearum [89]. In an oxygen-deficient environment, bacteria emit various volatile inorganic metabolites such as hydrogen sulfide and hydrogen. These compounds act as a precursor for amino acid, antimicrobial metabolites synthesis, or act as electron acceptors.
B. subtilis produce hydrogen sulfide from sulfate hydrolysis or a by-product of L-cysteine and L-methionine catabolism
[90][91].
6.2. Volatile Organic Metabolites
6.2.1. Terpenes and Terpenoids
Terpenoids, also known as isoprenoids, are widely produced by all living organisms
[92]. The end product of the deoxy xylulose phosphate pathway, isopentenyl pyrophosphate (IPP), and their isomer dimethylallyl pyrophosphate (DMAPP) usually act as precursors for terpenoid biosynthesis
[90]. However, terpenoids may also be synthesized from isoprene
[93]. As previously shown, the isoprene produced by
B. subtilis is not synthesized via the deoxy-xylulose phosphate pathway. Isoprenoid plays a vital role in physiological functions such as membrane fluidity, electron transport, light-harvesting, and cell signaling
[94]. The involvement of terpenoids in cell signaling is particularly important, as it is associated with some mutualistic, antagonistic, and multitrophic interactions
[95]. Isoprenoids may potentially be used as a flavor, nutraceuticals, fragrance, and therapeutic agent in malaria and cancer treatment
[96].
6.2.2. Nitrogen-Containing Metabolites
Nitrogen-containing metabolites can be distinguished based on their degree of cyclization. To date, three groups of non-cyclic (amine, amide, and imines) and five groups of cyclic compounds (pyrazines, azole, pyridines, pyrimidines, and pyridazines) have been detected. Pyrazines are broadly represented among mVOCs and are categorized into two subclasses: higher alkylated and lower alkylated pyrazines
[90]. These metabolites are characterized by a strong odor, and most of the
B. subtilis strains isolated from rhizosphere and fermented products have been considered as pyrazine producers
[97][98]. The compound 2,5-Dimethyl pyrazine was isolated from
B. subtilis strain, exhibiting strong antifungal activity and inhibiting
P. chlamydospora [99]. Pyrazine produced by B. subtilis strains also inhibits the growth of bacteria such as
E. coli,
S. aureus, and
P. valgaris [100].
6.2.3. Sulfur-Containing Metabolites
Sulfur-containing volatile organic metabolites are synthesized from two primary sources, i.e. organic or inorganic
[90]. These metabolites often originate from the catabolism of amino acids, such as methionine, and sometimes from cysteine. However, the inorganic sulfate and sulfite may also act as a precursor for sulfur metabolites. The
B. subtilis group produces numerous antifungal and antinematicidal sulfur metabolites such as S-methyl butanethioate, S-methyl thioacetate, 2-methyl disulfide, and 3-methyl trisulfide. Among these, dimethyl disulfide also exhibits antibacterial activity
[101][102][103][104]. It is known that dimethyl sulfide disrupts bacterial cell communication by decreasing the sum of acyl-homoserine lactone
[105].
6.2.4. Benzenoids
Benzenoids are a diverse subclass often linked with sulfur or/and nitrogen. Most of the benzenoids produced by the B. subtilis group displayed antifungal activity, while some inhibited the growth of bacteria and nematodes. Their antimicrobial mode of action is partially characterized. Nevertheless, the fungal and bacterial cell disruption is documented after exposure to bVOCs.
The
B. subtilis strain CF-3 was evaluated for their volatile organic metabolites potential. The investigator identified a plethora of volatile compounds based on solid headspace microextraction. Among them, benzothiazole exhibiting strong antimicrobial activity against fruit fungal pathogens
[106]. The volatile organic compounds produced by
B. amyloliqueficiens were evaluated for their effect on the growth and pathogenicity of tomato bacterial pathogen
R. solanacearum. The results showed that the strain emits several volatile organic compounds, including 1, 3 dimethoxy benzenes, inhibiting 62–85% growth of the tomato pathogen
[107].
6.2.5. Ketones
The biosynthesis of ketones is usually the result of fatty acid decarboxylation. Acetoin and its oxidative form butanedion are synthesized during the anaerobic fermentation of pyruvate. The two pyruvate molecules are condensed and converted to acetolactate by the acetolactate synthase enzyme. The acetolactate is further decarboxylated to form acetoin. Ketones are mainly known to inhibit the growth of plant pathogenic fungi. However, its antibacterial activity is yet not reported. Two ketones metabolites, i.e., 2-decanone, and 2-nonanone, displayed 100% growth inhibition of
F. oxysporum [108].
6.2.6. Hydrocarbon Metabolites
Hydrocarbon metabolites include alkane, alkene, and alkyne metabolites usually derived from fatty acid degradation via elongation decarboxylation or head-to-head condensation. Hydrocarbons are the most stable volatile organic metabolites and tend to remain in their original architecture over a long period of time. They may be used as a biomarker to estimate the age of primeval bacteria
[109]. The
B. subtilis group secretes various types of hydrocarbons such as nonane, tridecane, tetradecane, 2-methylpropane, and cyclohexane. Hydrocarbons like alkane, alkene, nonane, and decane are gaining particular interest due to their use as antimicrobial agents and fossil fuels. Nonane and 8-methyl heptadecane were isolated from
B. velezensis and exhibited antifungal activity against several fungal pathogens
[110]. Likewise, 1 3-butadiene inhibits the growth of phytopathogen and, additionally, negatively influences the chemotoxicity of the fungal pathogens
[111].
7. Miscellaneous Metabolites
There are few bioactive metabolites produced by B. subtilis that do not fit into any class. For instance, bacilysocin is neither synthesized via NRPS nor PKS. Instead, it is a phospholipid antibiotic that accumulates within the B. subtilis cell and presents a unique example of a modified phospholipid. Bacilysocin’s structure is composed of a central glycerol linked with glyceryl phosphate and an anteiso-fatty acid tail. The putative biosynthetic pathway for the bacilysocin initiate is from the conversion of phosphatidic acid to phosphatidylglycerol and then lysophospholipase (YtpA) convert phosphatidylglycerol to bacilysocin. The antimicrobial activity of bacilysocin is limited to Gram-positive bacteria and a few fungal strains, including S. aureus, Candida pseudotropicalis and S. cerevisiae. To date, the biological role, absolute biosynthetic pathway, mode of action, and the specific activity of bacilysocin is unclear.