Recently, extensive research has been classified into different types of free radicals. The three main categories are: reactive oxygen species (ROS), reactive nitrogen species (RNS), and reactive sulfur species (RSS), which are formed from oxygen, nitrogen, and sulfur atoms, respectively
[6]. Examples of ROS, RNS, and RSS include hydrogen oxide, hydrogen peroxide, singlet oxygen, alko-xyradical, peroxyl-radical, nitrogen monoxide, nitric oxide superoxide anion, hydroxyl anions, alkyl-thiol, etc.
[2][6][7]. More specifically, the ROS group includes lipid peroxidation products and protein carbonyl species while the RNS group includes nitric oxide and peroxynitrites. Nitric oxide plays a key role in DNA damage, inflammation, cancer cell growth, and apoptotic malfunction, even though it has a lifespan of only a fraction of a second. In addition, peroxynitrites have the potential to cause lipid peroxidation, DNA damage, and long-term damage to all biomolecules. Similarly, sulfur species (RSS) may act in unison to damage biomolecules and, hence, extensive damage to genes in DNA may result in genes that produce ineffective proteins
[4][8]. The origin of radicals is not yet well defined, but our own body often produces free radicals in the process of breaking down nutrients to create the energy that allows our bodies to function. Endogenous sources are multifaction in mitochondria, peroxisomes, endoplasmic reticulum, phagocytic cells, etc. while some exogenous sources may be air pollution, ultraviolet radiation, alcohol, smoking, contact with heavy metals, pesticides, and certain drugs such as halothane and paracetamol
[9].
The main representatives of antioxidants are vitamins A, C, and E; beta-carotene; anthocyanidins; phenols; flavonoids; phenolic acids, etc.
[17]. Certainly, natural antioxidants that are consumed daily in our diet can protect our bodies and act as anticarcinogenic agents. Higher antioxidant and anticancer activities are also demonstrated in cases where there is a synergetic effect between different antioxidant natural molecules
[18][19][20]. Endogenous defenses in humans have gradually improved over time, resulting in a balance between free radicals and oxidative stress. Enzymatic antioxidants and non-enzymatic oxidants are the two main types of antioxidants found in humans
[6]. Antioxidant defense mechanisms attempt to scavenge reactive oxygen species and prevent their formation, although they are not always successful. The antioxidant network is complex, containing substances that are both endogenous and ingested. Enzymes called superoxide dismutase (SOD) convert O
2 to H
2O
2 and eliminate it from the body
[21]. However, because of the blood–brain barrier, antioxidants sometimes fail to provide adequate protection
[22]. Numerous studies have demonstrated the necessity of antioxidants, but currently, the preferred choice of determination method is a controversial challenge. Antibody, fluorescence, light emission, spectrophotometric, chromatography, and electrophoretic techniques are the most widely used quantification procedures for determining the total antioxidant activity (TAA)
[6][23][24].
2. Oxidation Process and Radicals
As mentioned before, free radicals are chemical entities (atoms, molecules, or ions), that have a single unpaired electron in one of their outer orbits, which makes them unstable and reactive
[25]. The outcome is the formation of more radicals or unwanted side products as an electron attempts to bind with another. The majority have a half-life that depends on their environment. For example, the half-life of NO• or most oxygen species is a few minutes whereas the half-life of sulfur anion free radical is seconds. The initiation of the generation of radicals is any source of heat, ultraviolet irradiation, and air pollution, or naturally occurs in mitochondria. In humans, small molecules, peptides, proteins, and enzymes mostly contain nitrogen, oxygen, and sulfur, which serve a variety of functions in living creatures
[26]. Nitrogen and oxygen are usually bonded in ‘chains’ of two to three atoms (peroxides, ozone, dinitrogen trioxide, etc.) while sulfur chains can be considerably longer (
Table 1). These atoms have many oxidation states, and sometimes, during certain events and under certain circumstances, they release free radicals as side products
[2][25][26]. During the propagation step, free radicals react with other molecules until their termination, where the free radicals bind together in a way that the chain is no longer propagated.
Table 1. Reactive oxygen (ROS), nitrogen (RNS), and sulfur (RSS) species and their non-free-radical species.
Reactive oxygen (ROS), nitrogen (RNS), and sulfur (RSS) species are formed both endogenously and exogenously
[2]. The most prevalent free radicals that cause harm to biological systems are oxygen-free radicals, also known as ROS
[27]. They are produced as a by-product of biochemical reactions by neutrophils and macrophages in mitochondria, peroxisomes, and other organelles
[28]. Activated forms of ROS are illustrated in
Table 1 and are usually separated as small particles that do not contain carbon atoms, such as •OH, in contrast with forms such as ROO•. Reactive sulfur species have also received attention for their role in oxidative stress, a phenomenon caused by an imbalance between the production and accumulation of reactive species in cells and tissues and the ability of a biological system to detoxify these reactive products
[29]. Normally, sulfur radicals can be produced by hydrogen donation, enzymatic oxidation, and interaction with reactive oxygen species such as hydrogen peroxide, singlet oxygen, peroxynitrite, and superoxide
[26]. When cellular thiols are oxidized, they form species that can oxidize and inhibit the action of proteins and enzymes. Currently, known sulfur-free radicals include disulfides and monosulifes, disulfide-S-oxides, and sulfenic acid agents (
Table 1). According to Abedinzadeh et al.
[30], the formation of highly reactive sulfur radicals participates in several different reactions, which lead to disulfide radical anion, thiyl peroxyl radical, and others. On the other hand, reactive nitrogen species (RNS) are a class of antimicrobial compounds produced by nitric oxide and superoxide. When they combine, they are converted to a peroxynitrite free radical. Rapid protonation of peroxynitrite anion in vivo gives peroxynitrous acid (ONOOH), which acts as an electrophilic nitrating agent for tyrosine and tryptophan sidechains in proteins. The decomposition of peroxynitrous acid can generate hydroxyl radicals, which can subsequently damage human DNA
[31]. Further damage to DNA clones has been reported as the presence of nitric oxide free radical is related to dose-dependent DNA strand breaks and the transformation from cytosine to uracil and 5-methylcytosine to thymine
[32]. The endogenous antioxidant defense system can also be overwhelmed by ROS, RNS, and RSS, resulting in cellular damage and dysfunction, which leads to a variety of illnesses. ROS and RNS are key regulatory mediators in signaling pathways at low concentrations, but they are toxic in moderate and high quantities, inactivating critical cellular components
[33].
Nitric oxide (NO•) has two purposes in health and sickness and its level influences both. Nitric oxide has the potential to act as an active marker of cancer progression during physiological and pathological processes by encouraging angiogenesis or the production of new blood vessels
[34]. Furthermore, by upregulating p53, poly (ADP-ribose) polymerase, and DNA-dependent protein kinase, NO• may affect tumor DNA repair processes (DNA-PK). The use of NO• in cancer research has significant therapeutic implications for disease detection and treatment. At the same time, the ratio of ROS/RNS is engaged in a range of physiological activities, including immunological function (i.e., protection against harmful microorganisms), cellular signaling pathways, mitogenic response, and redox regulation, and has beneficial effects at moderate or low levels. However, at higher ratios of ROS/RNS, oxidative and nitrosative stress can occur, which can destroy biomolecules as the antioxidant and oxidant levels are unbalanced
[9][35]. Increasingly more free radicals build up, causing extensive damage to macromolecules, including nucleic acids, proteins, and lipids.
Peroxidation of lipid products and protein carbonyls is only one of the side effects of ROS when nitric oxide and peroxynitrites are produced from nitrogen radicals
[4]. Therefore, any anomalies that occur in these crucial structural components have been related to the onset of a variety of neurodegenerative diseases, such as Alzheimer’s, Parkinson’s, etc.
[36]. A study by Porter et al.
[37] indicated that malondialdehyde (MDA, ROS agent) interacts with low-density lipoproteins and, as a result, lipid peroxidation forms, which indirectly causes atherosclerosis. Additionally, ONOO
− is another major RNS player that acts as a lipid peroxidation catalyst, which causes membrane and lipoprotein disruption. In the growth of cancer, ONOO
− and MDA act as cytotoxic and mutagenic agents, promoting DNA damage through mutations, resulting in decreased tumor suppressor gene expression or enhanced oncogene expression
[38]. The possible consequences of the effects of lipids and proteins include tissue damage, neurological illnesses, cancer, cardiovascular diseases, cataracts, rheumatoid arthritis, asthma, stroke, myocardial infarction, chronic heart failure, diabetes, and many other neurodegenerative disorders
[4][22][31].
3. Classification of Natural Antioxidants
Antioxidants can be separated into two main categories: synthetic and natural, which are derivatives of fruits, herbs, and plants
[39][40][41]. Additionally, different plant by-products are also an economic source of natural antioxidants
[42]. Nowadays, the employment of synthetic antioxidants in food, such as butylated hydroxytoluene (BHT) or butylated hydroxyanisole (BHA), has raised social concern, as these compounds are effective and relatively cheap to produce, but they can generate allergies and serious problems for human health in the long term
[43][44]. This is also the major reason why many companies are trying to replace compounds synthesized in the laboratory with natural antioxidants to prevent oxidation in food products and secure a healthier lifestyle
[45]. However, antioxidants must meet some of the following criteria to be utilized in this task. Firstly, they must be efficient, and their utilization must be cost-effective. Secondly, large-scale usage is not practicable if they are too expensive or complex to synthesize or isolate. Finally, they need to be kept at low concentrations, as these substances can be harmful to people at extremely high levels.
It is well known that plants, fruits, vegetables, herbs, seeds, and other natural sources present a large cocktail of antioxidants, such as phenolic compounds, carotenoids, and vitamins
[16]. Due to this fact, a diet rich in fruits and vegetables is usually recommended in order to receive all the benefits. By doing so, it is possible to prevent or delay certain diseases, such as cardiovascular diseases or diabetes
[43][46]. This variety of phenolic acids and flavonoids, along with a general classification of antioxidants, is shown in
Figure 1. Additionally, some by-products of the food and agricultural industries, such as shells or peels, have been and continue to be investigated for the extraction of antioxidants. It is also important to note that, depending on the type of the plant and its morphological parts, the antioxidant capacity can vary significantly, as the antioxidant capacity of the leaves is not the same as that of the stem
[47]. Based on this, different processes such as extraction, separation, and characterization of natural antioxidants have been investigated over the years.
Figure 1. Classification of natural antioxidants
[43].
According to
Figure 1, natural antioxidants can be classified into endogenous and exogenous antioxidants. Endogenous antioxidants are synthesized internally by the metabolism while exogenous antioxidants are obtained mostly in plants. The combination of endogenous and exogenous antioxidants in the human body helps maintain the nucleophilic tone, which translates into a healthy physical state
[46]. Endogenous antioxidants can be divided into enzymatic and non-enzymatic. Enzymatic antioxidants act as the first line of defense in the human body while non-enzymatic antioxidants usually act as the second line of defense. The main representatives of enzymatic antioxidants with the highest effectiveness are superoxide dismutase (SOD) and catalase (CAT). SOD is responsible for obtaining O
2 and H
2O
2 from the O
2− radical. Then, CAT takes H
2O
2 and converts it to H
2O and O
2 [48]. Non-enzymatic proteins such as albumin and transferrin are also endogenous antioxidants. Proteins of this type are capable of trapping metal ions, avoiding the formation of new reactive species
[46]. Exogenous antioxidants can also be divided into many different compounds. The most significant ones that are present in the diet of an average person are phenolic compounds such as flavonoids and phenolic acids, vitamins such as ascorbic acid (C) and tocopherol (E), and carotenoids
[44].
In the case of vitamins, those that are water-soluble, such as ascorbic acid, are responsible for stopping free radicals present in the aqueous phase. Fat-soluble vitamins, such as tocopherol, are present in cell membranes, preventing their degradation
[48]. The largest groups of exogenous natural antioxidants are phenolic structures. Within them, a distinction can be made between phenolic acids and flavonoids. Both are present in plants and this is also the reason why plant-derived foods contain a large amount of these exogenous antioxidants. As can be seen in
Figure 1, these acids are divided into two groups depending on whether they are formed from benzoic or cinnamic acid. Some examples are caffeic acid (derived from hydroxycinnamic acid) and vanillic acid (derived from hydroxybenzoic acid). It should be noted that compounds derived from hydroxybenzoic acid have a lower antioxidant capacity than those derived from hydroxycinnamic acid. In general, it is estimated that the average person consumes about 200 mg/day of phenolic acid during the day
[43].
On the other hand, flavonoids are present in large amounts in plants, formed from primary metabolites. Plants can transform the amino acids tyrosine and phenylalanine into new compounds. All of them have a general structure consisting of 3 phenyl rings and another heterocyclic ring containing an oxygen atom, forming a 15-carbon skeleton. Many of these compounds with variations in the two phenyl rings are found in nature
[49]. Flavonoids are also present in the diets of people, reaching higher levels of daily intake than phenolic acids. Delving a little deeper into this group of compounds, flavonols are more common than flavones. They accumulate in the leaves and skin of plants and can act as complex-forming agents with metal ions due to the presence of carbonyl and hydroxyl groups in their structure
[43].
4. Natural Antioxidant Mechanism in Radical Scavenging
Antioxidants have a differing capacity to stop the propagation of free radicals. The important factors influencing this are both the structure of the antioxidant and the structure of the compound to be oxidized, the presence of pro-oxidants, and the concentration of all of them. In addition, the region in the organism where all these substances are present and react together must be considered. There are various ways in which antioxidants carry out their work and there are numerous variables that can affect the antioxidant capacity
[48]. This section will show some of the known mechanisms used by natural antioxidants in dealing with the propagation of free radicals. As the number of natural antioxidants is very large, only a selection of the best-known and most important ones will be discussed.
Starting with phenolic acids, which are among the most abundant exogenous antioxidants, their ability to neutralize free radicals depends on several things, including the number of hydroxyl groups present on their aromatic ring, their position on it, and the presence of other substituents. As a general rule, the greater the substitution of the aromatic ring, the greater the difference in the antioxidant activity of these compounds. An aromatic ring that is unsubstituted cannot act as a hydrogen donor and therefore has a lower antioxidant capacity
[50][51]. There are many different mechanisms by which an antioxidant compound can perform its function. The first and most common is known as HAT or hydrogen-electron transfer. This mechanism is used not only by phenolic acids but by all antioxidants that have a labile hydrogen atom in their structure. These compounds give up their hydrogen to stabilize the radical. Once this is carried out, the antioxidant compound becomes a radical itself, but it can be stabilized and reach a state where it is harmless
[52]. A way of measuring how easily a hydrogen atom of an antioxidant compound can react with a free radical is the bond dissociation energy. The lower the value, the easier a hydrogen atom is transferred
[53]. An example of this mechanism is shown in
Figure 2.
Figure 2. Phenolic acid derivative neutralizing a free radical via HAT
[43].
Another mechanism widely used by phenolic acids is the so-called SET or single electron transfer. In this case, the antioxidant gives up an electron to the free radical to stabilize it in an anionic form as shown in
Figure 3 [43][52].
Figure 3. Phenolic acid derivative neutralizing a free radical via SET
[43][52].
The third and fourth mechanisms by which the various phenolic acids exert their antioxidant capacity are known as transition metal chelation and sequential proton loss electron transfer (SPLET). The metal chelation mechanism is the ability of certain antioxidants to chelate transition metals, preventing them from catalyzing reactions that produce free radicals inside an organism (
Figure 4). Some of these metals are Fe, Cu, and Mn
[52][54].
Figure 4. Phenolic acid derivative chelating Fe
3+ and Fe
2+ [43][52].
On the other hand, SPLET, or sequential proton loss electron transfer, occurs when the antioxidant compound donates a proton to a free radical and transforms into an anion, which subsequently donates an electron to stabilize itself
[52]. An example of this is shown in
Figure 5.
Figure 5. Phenolic acid derivative neutralizing a free radical via SPLET
[52].
In the previous section, it was pointed out that antioxidants derived directly from cinnamic acid have a higher antioxidant capacity than those derived from benzoic acid. This is due to the presence of the double bond of cinnamic acid, which can conjugate with the electron cloud of the aromatic ring, giving it a greater capacity to stabilize reactive species. Moreover, since the carbonyl group of cinnamic acid derivatives is distant from the aromatic ring, their antioxidant activity is higher than that of benzoic acid derivatives
[48]. Flavonoids, as phenolic structures, also present these mechanisms. Their ability to neutralize free radicals is influenced both by the type of catechol ring present in their structure and by the presence of hydroxyl groups. Additionally, their double bond plays a critical role, as it can be conjugated and provide greater structural stability
[43]. An example of a typical flavonoid structure is shown in
Figure 6.
Figure 6. Most common structure of flavonoids. R1-R5 can range from hydrogen atoms to hydroxyl or methoxy groups
[43].
Apart from phenolic structures, there are many other compounds in exogenous antioxidants. Among vitamins, tocopherol, or vitamin E, is a fat-soluble vitamin present in cell membranes. Although this compound has a phenolic structure, it is classified within the group of vitamins. There are, in turn, different classes of tocopherols depending on their structure. α-Tocopherol is the most abundant form of vitamin E in nature
[43][46]. This compound uses the SPLET mechanism to exert its antioxidant activity (
Figure 7).
Figure 7. α-Tocopherol neutralizing a free radical via SPLET
[43][46].
In the case of endogenous natural antioxidants, the non-enzymatic ones, such as transferrin and albumin, use the mechanism of metal chelation to trap metal ions as already mentioned. As for those that are enzymatic, such as SOD and CAT, they catalyze a series of reactions essential for the correct functioning of the organism
[55]:

5. Spectrophotometric Methods for Measuring Antioxidant Activity
Based on the previous discussion, it clear that it is critical to investigate the techniques that can be used to determine the total antioxidant activity (TAC) and total phenolic content (TPC). In this context, spectrophotometric (colorimetric and fluorescence) tests have received more attention as they are fast, reproducible, easy, and cheap
[6][48][56]. Colorimetric assays, which are the most famous, such as DPPH, FRAP, ABTS, etc., change their color due to an electronic transition in atoms or molecules. A change in the electronic transitions affects how much light is absorbed by the molecules, which in turn alters the color of the molecules. Numerous complexes enter an excitation state after receiving an electron. The production of brightly colored complexes is caused by the fact that the excitation energy needed for an electron to transition from one energy level to another frequently falls in the visible area of the electromagnetic spectrum. Because of the different types of donors and acceptors involved, the absorption wavelength of the transition bands is unique. Any wavelength from 400 to 750 nm is visible as red, orange, yellow, green, blue, and violet
[57]. All spectrophotometric methods are quantification techniques as a result of the regression line and regression equation of different concentrations of standards
[58]. Depending on each case, the antioxidant structure and properties and the solubility and partition coefficient dictate the prevailing mechanism in a given system and guide the selection of the optimum assay
[56][59].
Table 2 illustrates the spectrophotometric assays that will be addressed in more detail, along with their absorption maxima (λ
max), fundamental principle, and any observed color shifting.
Table 2. Spectrophotometric parameters of each assay.
Assay
|
nm
|
Principle of Method
|
Determination
|
Color Shifting
|
Reference
|
From
|
To
|
|
DPPH
|
515–520
|
Antioxidant reaction with free organic radicals
|
Colorimetry
|

|
[10][58][60]
|
Folin–Ciocalteu
|
760–765
|
The reductive capacity of antioxidants to determine the total phenolic content
|
Colorimetry
|
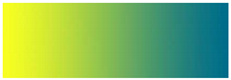
|
[58][61][62]
|
CUPRAC
|
450–490
|
Measures TAC of the reduction of Cu (II) to Cu (I) by antioxidants
|
Colorimetry
|
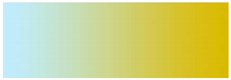
|
[48][63][64]
|
FRAP
|
593
|
Measures the antioxidant potential through the reduction of Fe (III) to Fe (II) by antioxidants
|
Colorimetry
|

|
[23][43][48][64]
|
ABTS
|
414, 645–650, 734, 815–820
|
Measures the relative ability of antioxidants to scavenge the ABTS generated in the aqueous phase
|
Colorimetry
|

|
[6][23][63][64][65][66]
|
ORAC and HORAC
|
485–525 and 485–535
|
Antioxidant reaction with peroxyl radicals and quench OH radicals generated by a Co(II)-based Fenton-like system
|
Loss of fluorescence of fluorescein
|

|
[5][24][48][65][67]
|
TBA-TBARS
|
532–535
|
Based on the reactivity of malondialdehyde (MDA) with TBA to produce a red color
|
Colorimetry
|
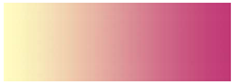
|
[23][68][69][70]
|
FOX
|
550–560
|
Measure the levels of hydrogen peroxide in biological systems by the oxidation of Fe(II) to Fe(III)
|
Colorimetry
|
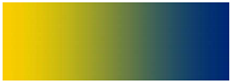
|
[23][71][72]
|
FTC
|
500
|
Measure the levels of hydrogen peroxide as the ferric ion is converted by an oxidant from a ferrous ion
|
Colorimetry
|

|
[23][70][73]
|
β-Carotene Bleaching Assay
|
440
|
Measure the levels of peroxyl radicals as β-carotene blenched
|
Colorimetry
|

|
[23][74]
|
Hydrogen peroxide scavenging
|
460
|
Total oxidant scavenging capacity of antioxidants
|
Fluorescence
|

|
[10][75]
|
Superoxide radical scavenging
|
560–562
|
Total oxidant scavenging capacity of antioxidants
|
Colorimetry
|

|
[75][76]
|
Nitric oxide radical scavenging
|
540
|
Total oxidant scavenging capacity of antioxidants
|
Colorimetry
|

|
[75]
|
Peroxynitrite Scavenging
|
485, 505, 529–530, 611
|
Total oxidant scavenging capacity of antioxidants
|
Fluorescence
|
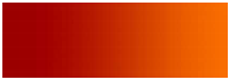
|
[77][78]
|
6. Advantages and Limitations of Spectrophotometric Assays
Antioxidant scavenging is becoming more interesting to scientists, who are attempting to understand the mechanisms involved in biological systems, the antioxidant capacity of food, and the free radicals of various substrates. Alternative methods for the measurement of antioxidant activity are still required (liquid and gas chromatography, electrophoretic procedures, etc.), although spectrophotometric applications are important. The benefits of employing spectrophotometric TAC assays are numerous and include their ease of use, low cost per sample, quick turnaround times, and the ability to be carried out manually, semi-automatically, or automatically. However, many parameters can affect the results of the measurements, such as the working pH area, the temperature of the current reaction, the applicability of the assay to both hydrophilic and lipophilic compounds, and others
[48][66][79][80]. Therefore, there is an imperative need to select the applicable assay in each case, as the ABTS and CUPRAC tests can detect both hydrophilic and lipophilic antioxidants and FRAP and FC exclusively detect hydrophilic antioxidants while others such as DPPH can only be applied to hydrophobic systems. At the same time, interferences that may appear could affect the color of the food matrix and can be absorbed in the same area as the antioxidants
[81].
The TAC tests have the benefit of being able to quantitatively assess the antioxidant components of a sample. It takes a lot of time and effort to measure each antioxidant component separately
[82]. Several studies on food, plants, and human body fluids have been the subject of several years of investigation. Cao et al.
[83] and Prior et al.
[59] observed no link between serum ORAC and TEAC or between serum FRAP and serum TEAC. Furthermore, to demonstrate how these approaches differ from one another, a comparative analysis of the antioxidant capacities of 30 plant extracts was performed using the DPPH, ABTS, and FRAP tests
[84]. The FRAP and ABTS assays had the highest correlation (0.946) while the ABTS and DPPH assays had the lowest correlation (0.906).
Undoubtedly, one of the most common assays is the DPPH approach. The application of this test facilitates an understanding of a variety of chemical processes and offers several obvious advantages, such as affordability, experiment simplicity, reproducibility, applicability at room temperature, and automation possibilities
[85]. However, the overlapping spectra of substances that are absorbed in the same wavelength range as DPPH is a significant drawback. For instance, anthocyanins exhibit significant absorption in the same wavelength range (500–550 nm) as DPPH, which could introduce interference into the data and affect how it is interpreted
[56]. On the other hand, CUPRAC reagent is more stable and convenient to use than other chromogenic reagents (e.g., ABTS, DPPH). The CUPRAC assay works best at a pH of 7.0, which is very similar to the physiological pH (7.4) and simulates antioxidant action in natural settings. Furthermore, it is characterized by robustness in contrast to free radical reagents, such as DPPH, as it is not affected by physiological conditions such as air, humidity, and sunshine
[48]. Additionally, CUBRAC, like the ABTS assay, is very selective because it has a lower redox potential than the Folin or ferric ion-based approaches. Additionally, the CUPRAC reagent does not cause oxidation of simple sugars or citric acid, which are not considered as real antioxidants, but the majority of phenolic antioxidants are readily oxidized due to their advantageous redox potentials. Moreover, the CUPRAC reagent can easily oxidize several antioxidants that are resistant to the FRAP, FOX, and FTC assays, with perfectly linear absorbance–concentration curves
[81]. Although, this assay does not assess antioxidant enzymes or certain thiol antioxidants, such as glutathione
[48][63].
Another favorable assay is the Folin–Ciocalteu test. Numerous benefits also exist for the use of FC to quantify TPC, including its ease of use, repeatability, and robustness. In fact, according to a previous report, there is a strong correlation in the Folin-determined concentration between FRAP and ABTS assays (0.946) in contrast with ABTS and DPPH assays (0.906)
[17]. It does, however, have significant shortcomings. This test is sensitive to pH, temperature, and the reaction duration. Therefore, careful selection of the reaction state is crucial for achieving consistent and trustworthy findings. Due to the involvement of non-phenolic reducing agents present in the system when reducing the Folin–Ciocalteu reagent, TPC overestimation is a significant concern for the Folin–Ciocalteu test. Reducing sugars and certain amino acids are some of these pollutants
[85].
Indirect measures, which are based on determining a sample’s capability to reduce a metal complex, can also be performed with FRAP. One major limitation of the FRAP assay is that an aqueous testing apparatus is required, but it provides quick, repeatable findings. Consequently, a water-soluble antioxidant must be used as the reference
[23]. In addition, the propensity of blue to precipitate, form a suspension, and stain the measurement vat is a drawback of this FRAP test. Because of this, the timing of the addition of Fe
3+ (FeCl
3) is crucial and may lead to inaccuracies in the interpretation of the outcomes
[48]. In fact, FRAP results can vary tremendously depending on the timescale of analysis. Moreover, after several hours of reaction time, the absorption of polyphenols such as caffeic acid, tannic acid, ferulic acid, ascorbic acid, and quercetin gradually increases. Some polyphenols have slower reactions and need more time to be detected while others react rapidly with iron complexes, leading to degradation into other compounds with differing or lower reactivity
[59]. Therefore, a single-point absorption terminus might not be indicative of a finished reaction. Regarding its limitations, any substance that has a lower redox potential than the redox pair Fe
3+/Fe
2+ has the ability to reduce this system, raising the FRAP value and producing artificially high findings
[59].
The FRAP assay has many similarities with the TEAC procedure, with the exception that TEAC is carried out at neutral pH and the FRAP assay is performed under acidic (pH 3.6) conditions
[24]. The main advantage of TEAC is that it uses ABTS, which is soluble in both aqueous and organic solvent environments, allowing simultaneous assessment of hydrophilic and lipophilic antioxidants. Since the ABTS radical scavenging method can be tested over a wide pH range, it is useful for researching how pH affects antioxidant mechanisms in food-related components. Furthermore, this simplifies operations and allows for automated analysis. On the other hand, it could take a while to reach an endpoint due to the radical ABTS employed in the procedure, which does not reflect a physiological radical source. Although, due to the use of the synthetic ABTS radical cation, which is not present in food or biological systems, the TEAC assay has also been criticized for lacking biological relevance. As a result, numerous phenolic substances can interact with ABTS
•+ because they have low redox potentials
[48][81]. A previous study also suggests that there is no correlation between the HORAC and ORAC values
[86]. ORAC measures the capability of absorbing peroxyl radicals while HORAC principally measures the ability to prevent metal-chelating radicals from doing so. Samples with high HORAC values are therefore anticipated to not necessarily have high ORAC values and vice versa. Due to the fact that many antioxidants are also metal chelators, the Fe(II)/H
2O
2 mixture suffers in a scavenging assay. This is also the reason why FC has replaced ORAC in many cases
[77]. It is therefore impossible to determine whether the antioxidants are merely effective metal chelators or HO• scavengers. By converting Fe(III) to Fe(II), dietary antioxidants (such as vitamin C) can function as pro-oxidants and increase the rate of oxidation
[24].
Finally, the TRAP, TBARS-TBA, and β-carotene bleaching assays are used for their applicability to many different carbonyl compounds formed from lipid peroxidation. Generally, there is a good correlation between the FOX and TBARS approaches. However, in the study conducted by DeLong et al.
[71], the UV-induced increases were greater in TBARS plant tissues than in the FOX assay. In a previous survey by Bhuvaneswari et al.
[87], FTC was used to evaluate the total phenolic content, flavonoid content, and antioxidant properties among different cultivars of
Piper betle L. In comparison with other assays, no significant difference was found between ABTS and FOX while FOX was also as good as TBA and FRAP
[87]. Moreover, the TRAP values for a given combination of antioxidant compounds are often lower than the TEAC values while the correlation between FRAP measurements is typically low
[59][88]. Meanwhile, the TRAP and HORAC correlation coefficient is mentioned as 0.94 while in the case of ORAC-TRAP, the correlation is found to be r = 0.96
[88]. However, one major disadvantage of the TBA assay is that is not specific to MA, and this results in an overestimation of the MA concentration
[79]. Additionally, due to the instability of the substrates utilized for lipid peroxidation, antioxidant assays based on the spectrophotometric methods of thiobarbituric acid-reactive substance production have low reproducibility. Finally, the bleaching β-carotene test is disadvantaged by its inability to be repeated, as the complexity of the reaction involving carotenes under oxygen shows antioxidant action at low oxygen concentrations and propagation of the oxidative chain in air-saturated solutions
[89].