The highly transmittable and infectious COVID-19 remains a major threat worldwide, with the elderly and comorbid individuals being the most vulnerable. While vaccines are currently available, therapeutic drugs will help ease the viral outbreak and prevent serious health outcomes. Epigenetic modifications regulate gene expression through changes in chromatin structure and have been linked to viral pathophysiology. Since epigenetic modifications contribute to the life cycle of the virus and host immune responses to infection, epigenetic drugs are promising treatment targets to ameliorate COVID-19. Deficiency of the multifunctional secosteroid hormone vitamin D is a global health threat. Vitamin D and its receptor function to regulate genes involved in immunity, apoptosis, proliferation, differentiation, and inflammation. Amassed evidence also indicates the biological relations of vitamin D with reduced disease risk, while its receptor can be modulated by epigenetic mechanisms. The immunomodulatory effects of vitamin D suggest a role for vitamin D as a COVID-19 therapeutic agent.
1. Introduction
Coronavirus disease 2019 (COVID-19), caused by Severe Acute Respiratory Syndrome Coronavirus 2 (SARS-CoV-2), is still a major focus of research as no definitive treatment has been found. Its rapid spread and mutational rate emphasize the importance of fully understanding the viral structure and its biological function. The SARS-CoV-2 spike protein has been demonstrated to attach to the angiotensin-converting enzyme 2 (ACE2) receptor on the cell surface, which is expressed in the lungs, kidneys, immune cells, vascular endothelia, small intestine epithelial cells, and testes
[1][2]. Upon attaching to the host ACE2, viral entry is mediated by an endosome or spike glycoprotein cleavage through host cell proteases such as furin or transmembrane protease serine 2 (TMPRSS2). The viral RNA polymerase then aids replication and transcription of the virus in the cytoplasm, while the host ribosome machinery mediates protein synthesis of the viral RNA. Finally, the virion is assembled in the host’s endoplasmic reticulum-Golgi intermediate complex, enabling the mature virus to integrate into small smooth-walled vesicles and be secreted by the host cells
[3].
Upon detection of infection, several host cells attempt to stop or slow viral replication through significant alterations, such as prompting the innate and adaptive immune responses, including cell death in extreme cases. Over time, viruses have co-advanced with their host to develop independent mechanisms to fight or escape the antiviral cellular responses
[4]. These mechanisms may induce the reprogramming of the host cell to generate a favorable atmosphere for viral replication, thereby influencing infected cells to become virus-producing factories. The host cell competes against the virus and attempts to constrain it, while the virus counteracts and manipulates the host cell for its own benefit. During this battle, several viral infections initiate epigenetic modifications. Epigenetic modifications control antiviral gene expression as well as the expressions of host cell features employed by the virus for competent replication and transmission
[4]. Epigenetic modifications also enable cells to react to environmental deviations and adapt to environmental stimuli via alterations in gene expression profiles.
The heritable epigenetic mark, DNA methylation, occurs at sites rich in CpG dinucleotide repeats, identified as CpG islands. DNA methylation is characterized by the incorporation of a methyl group to the C-5 position of DNA’s cytosine ring, forming 5-methylcytosine. This addition is facilitated by DNA methyltransferase (DNMTs) enzymes in an S-adenosylmethionine-dependent reaction. Studies suggest that five families of DNMTs exist, including DNMT1, DNMT2, DNMT3A, DNMT3B, and DNMT3L
[5]. DNA methylation determines the expression of genes via hypomethylation, which results in the activation of gene expression, and hypermethylation, which leads to silenced gene expression
[6]. Recent research has linked DNA methylation with
ACE2 gene regulation. Accordingly, increased ACE2 expression within sparsely methylated CpG islands in the lungs, liver, and brain renders these organs more vulnerable to COVID-19 damage
[4].
Vitamin D controls 3% of the human genome
[7]. Vitamin D is acquired from the diet and/or synthesized in the skin’s epidermal layer in the presence of UV from sunlight
[8]. Vitamin D is widely recognized for its involvement in bone formation; however, emerging evidence has indicated its involvement in other processes, such as immune regulation
[9]. Vitamin D targets numerous immune cells such as monocytes, macrophages, dendritic cells, T-lymphocytes, and B-lymphocytes, thus emphasizing its role in both innate and adaptive immunity
[9]. These immune cells exhibit vitamin D activating enzymes which convert the inactive vitamin D to its active form, 1,25-dihydroxyvitamin D3, within the immune system; 1,25-dihydroxyvitamin D3 is involved in maintaining immune cell homeostasis
[9]. Vitamin D may also aid the host’s defense against viral infections by influencing the expression of toll-like receptors (TLRs) and regulating beta-defensins which cleave the viral membrane
[10]. In cases of viral infection, vitamin D impacts the immune system through lysosomal enzyme activation and nitric oxide release, which helps combat infection
[11].
2. Epigenetics and COVID-19 Infection
It is well known that viruses employ epigenetic processes, particularly CpG methylation, to undergo endocytosis and syncytium formation. During viral progression, the virus fuses with the cell membrane of the host and/or induces host cell-cell fusion. Both these processes promote virus endocytosis, along with the invasion of neighboring cells and innate antiviral immune system evasion
[12]. A syncytium, formed during membrane-virus or cell-cell fusion, is generally associated with CoVs, with SARS-CoV-2 being no exemption
[13]. Syncytin genes are hypomethylated and highly active in the mammalian placenta, while they are hypermethylated and silenced in other tissues, imposing risks to various diseases
[14]. Epigenetics has provided information on developmental biology, heritability, and memory techniques, with research showing more relevance in cancer, immunity, and infectious diseases
[15]. Epigenetic research has revealed that DNA and RNA viruses develop antagonizing regulatory roles via host metabolism and gene expression alterations, thereby creating an environment permissive to the spread and replication of the virus
[16]. CoVs facilitate epigenetic modifications by antagonizing the host’s presentation of the antigen or by antagonizing the activation of interferon-response genes
[17]. In addition, age-related host epigenome modifications may hinder the function and configuration of immune cells, thus influencing viral defenses and the adaptive immunity
[18]. This effect of the epigenome may explain the vulnerability of the elderly to SARS-CoV-2 infection
[19]. It has also been demonstrated that the
ACE2 gene production rate is epigenetically regulated
[20]. Furthermore, based on the methylation patterns of multiple CpG islands, Corley and Ndhlovu
[21] showed that the
ACE2 gene functionality is linked to age and gender. The ACE2 receptor, expressed in a range of human organs and tissues, presents the lowest rate of methylation in lung epithelial cells, suggesting that the rate of ACE2 transcription and expression is the highest in the lung tissue
[21].
2.1. DNA Methylation
DNA methylation profile analysis of four different lung tissue public databases enabled the assessment of DNA methylation at two
ACE2 CpG sites. It was revealed that age-related DNA methylation in airway epithelial cells occurred in the proximity of the
ACE2 gene transcription start site. Utilizing the Illumina DNA methylation array, samples from diverse biological ages expressed hypomethylation during aging at a CpG (cg085599149) close to the
ACE2 transcription site (TSS200 region). Previous studies have also indicated that aging downregulates global DNA methylation, resulting in differential methylation patterns of aging, inflammatory, and immune response genes
[21]. Consistent with epigenetic aging, which results in certain genes becoming more active and other genes becoming less active
[22], increased lung tissue ACE2 expression in older people renders them more vulnerable to viral diseases, including COVID-19. In contrast, the
ACE2 gene in organs and tissues of children is typically hypermethylated and thus virtually silenced
[6]. This phenomenon may justify the enhanced sensitivity of the elderly to symptomatic SARS-CoV-2 infection in contrast to younger individuals
[23].
2.2. Histone Modifications
Severe COVID-19 cases have been correlated with high lung ACE2 expression in patients with comorbidities. Experimental and network analysis from 700 human lung transcriptomes with high
ACE2 expression and underlying conditions identified histone acetyltransferase 1 (HAT1), histone deacetylase 2 (HDAC2), and lysine demethylase 5B (KDM5B) as prospective regulators of
ACE2 [23]. HDAC2 plays a role in immune responses against viruses
[24] and contains a cleavage site that is possibly targeted by the SARS-CoV-2 non-structural protein NSP5. Therefore, interactions with NSP5 may inhibit the HDAC2 nuclear localization
[4]. Pathway enrichment evaluation disclosed that
ACE2-related genes were controlled by KDM5B as well as specific histone methylation and acetylation marks, including mono-methylation (me) and trimethylation (me3) of lysine 4 (K4) on histone H3 and histone H3 acetylation of lysine 27 (H3K27ac). KDM5B regulates chromatin accessibility by repressing active chromatin marks, including di- and trimethylation of H3K4, which plays a role in DNA repair and gene transcription. Additionally, breast cancer patients demonstrated that KDM5B inhibition triggered an interferon response, resulting in resistance to DNA and RNA viral infections, thereby proposing a role for KDM5B as a COVID-19 prevention target
[23].
2.3. miRNAs
Over time, miRNAs have gained popularity as a novel tool for medical therapies
[25] and have been recognized as regulators of viral infections
[26]. Viral miRNAs encoded by SARS-CoV-2 can target multiple host genes. A study predicted 3377 target human genes as distinctive targets of 170 mature miRNAs from SAR-CoV-2. The spike protein and
open reading frames (ORF)
1ab are targeted by 67 and 369 different miRNAs, respectively, while 10 identified miRNAs contain binding sites across the SARS-CoV-2 genome
[27]. Farr, Rootes
[28] analyzed the circulating miRNA profiles in the plasma of COVID-19 patients. Differential expression in COVID-19 patients was observed in 50 miRNAs, of which 30 miRNAs were downregulated while 20 miRNAs were upregulated. Among these, miR-31-5p, miR-3125, and miR-4742-3p were the most upregulated candidates, while miR-1275, miR-3617-5p, and miR-500b-3p were the most downregulated of all miRNAs. Anti-inflammatory miR-766-3p showed the greatest statistically significant change. Farr, Rootes
[28] then determined whether miRNA profiles of COVID-19 patients could independently identify SARS-CoV-2 during early infection stages. Logistical regression analysis revealed that the measurement of mi-R195-5p showed a 90% accuracy, 95% precision, and 72% recall for COVID-19 identification, while a combination of miR-423-5p, miR-23a-3p, and miR-195-5p received 99.9% accuracy, 99.8% precision and 99.9% recall
[28].
3. Vitamin D Regulation
Active vitamin D, 1,25-dihydroxyvitamin D3, is synthesized from the 25-hydroxyvitamin D3 precursor via the 1α-hydroxylase enzyme (CYP27B1) (Figure 1). CYP27B1 is expressed in epithelia which forms the main barrier between the body and its environment. Upon invading pathogens, epithelia respond via its own innate immune system, activating macrophages and dendritic cells to recruit T cells and neutrophils to the infection site [29]. Therefore, during vitamin D insufficiency, reduced availability of the vitamin D precursor would impair active vitamin D production and subsequently alter the innate immune function [30]. Over time, vitamin D deficiency has surged worldwide [31] and has been connected to impaired lung function, enhanced inflammation, and poor immunity [32]. Ligand binding stimulates the translocation of the VDR from the cytosol to the nucleus, where the VDR heterodimerizes with the retinoid X receptor (RXR) to form the VDR/RXR complex. This complex attaches to specific genomic sequences on the promoter region of target genes, known as vitamin D response elements, thus activating or suppressing gene transcription on promoters by recruiting transcription factors and co-regulatory molecules [33]. Active vitamin D is vital for immune regulation. In contrast, vitamin D deficiency was previously associated with the pathogenesis of chronic lung diseases such as asthma and chronic obstructive pulmonary disease in populations exposed to airborne particulates [34]. Vitamin D enhances innate immunity via the antiviral peptide secretion [35], which enhances mucosal defenses. Dietary vitamin D controls genes involved in cell apoptosis, proliferation, differentiation, immune responses, and inflammation [36].
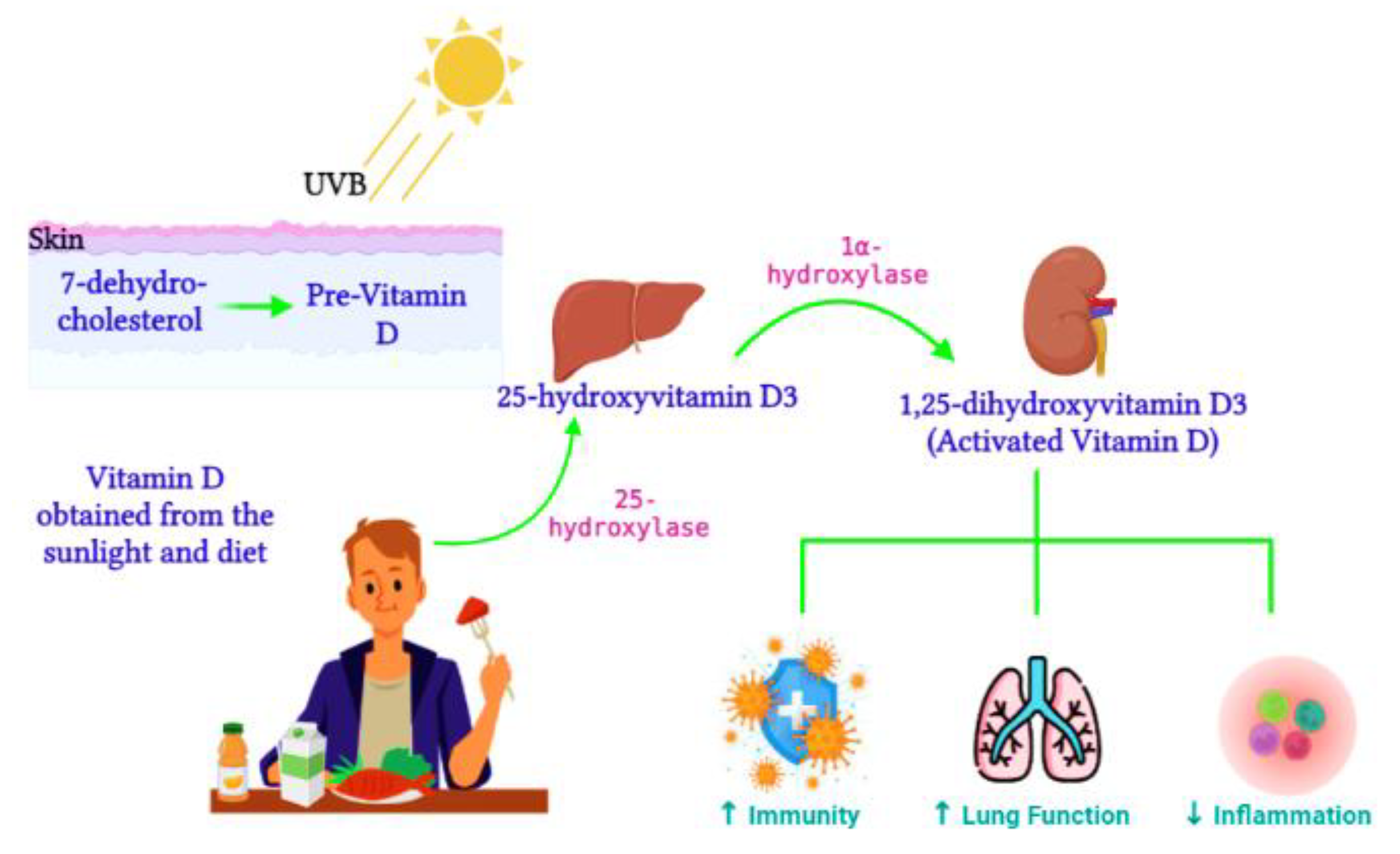
Figure 1. Vitamin D metabolism. During sunlight exposure, UVB radiation converts 7-dehydrocholesterol to pre-vitamin D in the skin. Vitamin D acquired from the diet t is converted by 25-hydroxylase to 25-hydroxyvitamin D3 in the liver. Activated vitamin D, 1,25-dihydroxyvitamin D3, is then synthesized in the kidney. Activated vitamin D improves immunity and lung function and reduces inflammation (Image created on Biorender.com).
3.1. The Impact of Vitamin D on Epigenetics and Gene Expression
Vitamin D was originally described as regulating calcium homeostasis; however, it now has crucial functions in various biological processes and diseases. Vitamin D metabolism promotes epigenetic modifications by influencing metabolic factors. While other vitamins (vitamin B12) and nutrients act as direct donors of methyl groups or inhibitors (e.g., resveratrol) of DNA promoter methylation
[37], the epigenetic mechanisms of vitamin D are induced mainly by its receptor VDR. This could also involve the expression of CYP27A1 and CYP27B1, which are key enzymes associated with converting Vitamin D3 to pre-hormones calcitriol and calcidiol and the CYP24A1 inactivating enzyme
[38]. Therefore, calcitriol and calcidiol both mediate vitamin D signaling to the VDR, which often builds dimers with retinoid X receptors
[39] and attaches to vitamin D response elements, thus influencing gene transcription. During transcription, the VDR/RXR dimers network with HATs, introducing acetyl groups to the nucleosomes and opening chromatin, thus activating the transcription
[40]. It is suggested that VDR promoter methylation may also establish a calcitriol insensitivity
[41]. VDR-mediated epigenetic regulation of microRNAs
[42] or tumor suppressor genes
[43] control the response of target promoter genes. Gene expression studies indicate that drugs under epigenetic activation potentially reverse calcitriol insensitivity. Genome-wide analysis revealed that VDRs typically attach at the loci of open chromatin, and upon 1,25-dihydroxyvitamin D3 liganded VDR treatment, chromatin accessibility of these loci is increased
[44]. The mechanisms of liganded VDR activity depend on the binding and activity of histone acetyltransferases and methyltransferases
[45]. Epigenetic corruption of VDR signaling leads to poor 1,25-dihydroxyvitamin D3 responsiveness, which may occur via promoter methylation of important vitamin D genes or by a skewed build-up of VDR-associated co-repressors, favorably at anti-proliferative target gene promoters
[46].
3.2. The Role of Vitamin D in COVID-19 Infections
The initial link between vitamin D and the immune system was brought about by the synthesis of active vitamin D from its precursor by antigen-presenting cells such as dendritic cells and macrophages. Active vitamin D, produced in the lungs, is vital for pulmonary immune responses. In airway epithelium, vitamin D controls VDR-induced gene expression to identify and eliminate pathogens through TLR co-repressor CD14 and antimicrobial peptide mechanisms
[47]. Additionally, in airway epithelium, NF-κβ-induced cytokine and chemokine expressions are modulated by 1,25-dihydroxyvitamin D during viral infections
[48]. To decrease the hazard of common colds, vitamin D utilizes three pathways: adaptive immunity, physical barrier, and natural cellular immunity. To minimize COVID-19 infection and mortality risk, vitamin D possibly functions in maintaining gap and cell junctions, reducing the cytokine storm, enhancing cellular immunity, and regulating adaptive immunity via responses of T helper cell type 1 and inducing T cells
[49]. In terms of COVID-19 infection, vitamin D promotes antiviral immunity by inducing defensins and cathelicidin that hinder viral entry and impairs viral replication
[50]. Vitamin D also promotes autophagy, an essential homeostasis mechanism involving the encapsulation of misfolded proteins and impaired organelles. Encapsulation of viral particles by autophagy packages these particles for lysosomal degradation, followed by antigen presentation and adaptive antiviral immune responses
[51]. Autophagy stimulation is a vital vitamin D cellular response as active vitamin D and its precursor both enhance the expression of the autophagy marker LC3
[52].