1. Abiotic Stress Adaptations
Plants often have to adapt to environments that are unfavorable for growth and development. Some plants acquired adaptive traits during evolution to deal with extreme environments, such as deserts, tundra, or swamps. To withstand these harsh environmental conditions, plants developed morphological and physiological adaptations, as well as signaling pathways that elicit biochemical and molecular mechanisms to survive different stress conditions
[1]. The stressful environmental factors can be either biotic or abiotic. Abiotic stresses mainly include drought, salinity, extreme temperatures, flooding, oxidative stress, nutrient deficiencies, and heavy metal stress
[2]. Drought is the major cause of crop losses around the world and water provision for agriculture was a key element for civilization’s success
[3][4]. Drought, salinity, cold, and freezing induce osmotic and oxidative stress and increase intracellular ion concentration, thus leading to reactive oxygen species (ROS) accumulation, protein denaturation, membrane disruption, and nucleic acid damage (
Figure 1).
Figure 1. Plant response mechanisms to abiotic stress; Abbreviations: ROS—reactive oxygen species; CAT—catalase; GR—glutathione reductase; SOD—superoxide dismutase; APX—ascorbate peroxidase.
At least three adaptive strategies have evolved in plants to live under drought conditions
[5]. Ephemeral plants are annual and have a short life cycle that allows them to grow and develop during the rainy season before forming seeds to escape unfavorable conditions. Another survival strategy is present in Cactaceae and Agavaceae plant families, which due to the presence of spikes as modified leaves, a long root system to capture as much water as possible, their stem/leaves sheltered with a thick waxy cuticle that covers a water-storage parenchyma, and adjusting their photosynthesis system with closed stomata and a C
4-carbon assimilation system, maintain the plant as a water reservoir to avoid water loss. The last group is true desiccation-tolerant plants, better known as resurrection plants, which can survive long periods of even decades without water to restart their metabolism and growth in a few hours after being again in contact with water (
Figure 1)
[6].
Plant desiccation-tolerance was a key trait to conquering land environments, although it is rare in vegetative tissues it is present in mature seeds but is lost during germination
[7]. Some genetic and biochemical components for drought tolerance are shared between seeds and resurrection plants and to some extent in non-tolerant plants displaying hardening or acclimation phenotypes
[8]. These latter plants require the expression of stress tolerance genes for a gradual adaptation to harsh conditions. Cultivated plant varieties are usually more sensitive to abiotic stress than their wild-type relatives because breeding selection for yield and plant size traits are not necessarily linked to stress tolerance genes.
A water deficit reduces photosynthesis by inducing stomata closure and inhibiting electron transport reactions and oxygen production, thus leading to ROS accumulation, which causes damage to the photosynthetic reaction centers
[9]. Plant adaptations to photosynthesis damage under drought stress are limited to a few plants containing C
4 carbon assimilation metabolism and crassulacean acid metabolism, which minimize photorespiration and a more efficient CO
2 harvest
[10]. Gene transfer experiments using transcription factors and Calvin-Cycle enzyme genes might soon improve photosynthesis and yield under abiotic stress conditions
[11][12].
Another important adaptation to water stress involves root architectural plasticity (
Figure 1). Plants with longer roots usually display increased yield since they have a higher water use efficiency measured as biomass production to water use ratio
[13]. During drought, ABA signals the SNAC1 transcription factor, which induces stomata closure, shoot growth arrest, and induces root growth, whereas lateral root growth is reduced due to the expression of the
MYB96 transcription factor and microRNA miRNA393
[14][15].
2. Physiological and Biochemical Responses
Protective mechanisms against abiotic stress include osmotic adjustment, antioxidant metabolism, and maintenance of cell membrane stability (
Figure 1)
[16]. Plant cells actively accumulate solutes when cellular dehydration occurs as part of osmotic adjustment
[17]. Metabolic-compatible compounds can accumulate in large quantities and are major drivers of osmotic adjustment under salt, drought, and cold stresses, helping with membrane stabilization, protection of the quaternary structure of proteins, and neutralization of toxic compounds under stressful conditions. It is the common strategy adopted by many organisms to combat environmental stress
[18]. These compatible compounds, known as osmoprotectants or osmolytes, are low molecular weight water-soluble compounds. The most common but not exclusive compatible solutes are sugars (sucrose and trehalose), polyols (mannitol and sorbitol), polyamines (putrescine, spermine, and spermidine), amino acids (glutamine and proline), and quaternary amines (glycine-betaine and choline-O-sufate)
[19]. For instance, spinach, sugar beet, and amaranth accumulate high levels of glycine-betaine, which is synthesized by two enzymatic steps. First, choline monooxygenase converts choline into betaine aldehyde, and then betaine aldehyde dehydrogenase synthesizes glycine-betaine
[20]. These enzymes are found in the chloroplast stroma. Proline is an osmolyte with an active role in plant growth and development in many plants, such as maize, rice, and legumes, and is synthesized in the cytoplasm through two major pathways. In the glutamate pathway, it is produced from glutamate by
Δ1-pyrroline-5-carboxylate synthetase and
Δ1-pyrroline-5-carboxylate reductase enzymes; in the ornithine pathway by ornithine-delta-aminotransferase, producing glutamic semialdehyde and
Δ1-pyrroline-5-carboxylate, which is converted to proline
[21]. Another important class of compatible solutes is sugar alcohols, also known as polyols, found in a wide range of species such as grapevine, apple, coffee, berries, and olives. Sorbitol and mannitol are synthesized by specific polyol dehydrogenases either from glucose-6-phosphate or mannose-6-phosphate, respectively, and protect cells against osmotic stress and metabolic imbalance between source and sink plant organs
[22].
A peculiar molecule is the disaccharide trehalose, which functions as an osmoprotectant against heat, salt, and drought stresses and is found at high concentrations in certain archaea and eubacteria species, some fungi and lower invertebrates, and desiccation-tolerant plants such as the lycophyte
Selaginella lepidophylla and in some rare Poaceae family members
[23]. There are five biosynthetic pathways, and the most common in several organisms, including plants, is a two-step process, where initially trehalose-6-phosphate synthase (TPS) condenses glucose-6-phosphate and UDP-glucose into trehalose-6-phosphate, which is converted to trehalose by trehalose-6-phosphate phosphatase (TPP). Interestingly, both TPS and TPP are also present in most plants and are encoded by multi-gene families; however, trehalose is almost undetectable
[24]. The intermediate compound trehalose-6-phosphate also acts as a signal molecule involved in growth, development, and crop yield
[25][26].
The non-toxic nitrogenous polycationic molecules named polyamines, usually bound to nucleic acids and proteins, accumulate in all organisms, and are involved in membrane stability and ROS scavenging
[27]. Some researchers consider polyamines as phytohormones since they participate in various plant functions, including regulating cell division, membrane and cell wall stabilization, growth, flower and fruit development, and adaptation to biotic and abiotic stresses
[28].
Oligosaccharides such as fructans, staquiose, and raffinose are involved in cold acclimation and freezing tolerance in a wide range of plants
[29]. For instance, in spite of lacking epidermal tissues,
Physcomirium patens moss displays freezing tolerance due to the accumulation of the trisaccharide theanderose
[30].
Major cultivated crops such as wheat, rice, and maize do not accumulate osmolytes at significant concentrations
[20]. However, it has been shown that the overexpression of proline, glycine-betaine, fructans, and trehalose biosynthetic genes leads to abiotic stress-tolerant phenotypes and plants with higher biomass and yield
[31]. In addition to their role as osmolytes, all these compounds are also involved in inhibiting ROS accumulation and protecting the photosynthetic apparatus.
Similar to osmotic adjustment, antioxidant defense systems are also important to stress tolerance mechanisms (
Figure 1). Drought, cold, heat, and salt stress induce the formation of reactive oxygen species (ROS) such as hydrogen peroxide (H
2O
2) and superoxide (O
2−)
[32]. These ROS are extremely toxic compounds, which can severely damage cell membranes, proteins, and DNA, eventually provoking cell death. In order to combat oxidative damage, plants utilize antioxidant defense mechanisms including enzymes such as superoxide dismutase (SOD), ascorbate peroxidase (APX), peroxidase (POD), catalase (CAT), and glutathione reductase (GR)
[33] (
Figure 1). In this regard, sugars also act as ROS quenchers and exert protective roles and stress tolerance when specific sugar types are localized in specific cellular compartments or in close vicinity membranes
[34]. Crops with enhanced ascorbate accumulation promote abiotic stress tolerance either by engineering increased biosynthesis, enhanced recycling, or modulating regulatory factors
[35].
Heat during summer days and at midday is a major stress that plants cope with by accumulating heat-shock proteins (HSP) to alleviate cellular damage (
Figure 1). HSPs are a broad protein family of molecular chaperones that comprises HSP100, HSP90, HSP70, HSP60, HSP40, and HSP20 that prevent and restore protein aggregation and misfolding and are present in bacteria and animals as well
[36]. Overexpression of different HSPs can partially alleviate heat stress in plants; however, engineered expression of upstream elements in the signaling pathway, as exemplified by the HSP transcription factor (HSF), which confers a better response, also induces tolerance to multiple stresses
[37].
As an additional tool to cope with abiotic stress, plants acquired a group of low molecular weight (10–30 kDa) proteins to protect against subcellular damage caused by drought, salt, or cold stress. These are named late embryogenesis abundant (LEA) proteins rich in glycine and lysine and have a hydrophilic and disordered nature, which under stress conditions reorders and forms repeated
α-helixed stretches (
Figure 1)
[38]. LEAs were originally found in mature seed embryos but, later on, were also identified in stressed vegetative tissues of most plants and even in some desiccation-tolerant invertebrates
[7][39]. They are classified into five families according to their protein similarity and are localized in the nuclei and cytoplasm, interacting with cellular proteins to prevent unfolding or stabilize membranes during osmotic stress
[38]. Either an ABA-dependent or an ABA-independent pathway can induce
LEA genes. For instance, two well-studied cases in Arabidopsis are the
RD29A and
RD29B LEAs protein genes. The
RD29B gene promoter has an ABA-regulated element, the ABRE box (CACGTG), whereas the
RD29A promoter has an ABA-independent dehydration-responsive element, the DRE box (TACCGACAT), induced by desiccation salt and cold stresses
[40]. The overexpression of the pepper CaLEA1 in Arabidopsis confers osmotic stress tolerance
[41].
Water transport along the plant from the soil into the roots is a key element for plant development and physiology (
Figure 1). Aquaporins function by regulating water trafficking through cell membranes in normal and stressful conditions but can also transport small solutes such as monosaccharides, polyols, silicon, and boron or gases such as ammonia or carbon dioxide
[42]. There are between 25 and 30 kDa proteins forming transmembrane tetramers and belonging to the major intrinsic protein (MIP) superfamily with members in most organisms
[43]. In plants, aquaporins are classified into seven subfamilies according to their sequence similarity, most of them plasma membrane intrinsic proteins (PIPs), tonoplast intrinsic proteins (TIPs), and nodulin 26-like intrinsic proteins (NIPs)
[44].
Together with drought, soil salinity is severely threatening agriculture since around 20% of total cultivated land and 50% of irrigated fields worldwide are affected by salinization
[45]. Salt stress inhibits plant growth and yield, germination, photosynthesis, and transpiration, and alters ion homeostasis (K
+/Na
+) caused by the combined effects of osmotic stress and ion toxicity, mainly due to Na
+ and Cl
− accumulation in the plant cytosol (
Figure 1)
[46]. Plant osmotic stress is dealt with the aid of osmoprotectants, and antioxidant molecules previously discussed. According to their response to salt stress, plants are classified as salt-tolerant or halophytes, and salt-sensitive or glycophytes
[47]. When Na
+ levels rise excessively in the plant, the K
+ uptake is inhibited, the latter being an essential ion for photosynthesis and metabolism as an enzyme cofactor. Hence, plant cells need to either extrude Na
+ from the cell, accumulate it on the vacuole, which also maintains cell turgor, or transport Na
+ to a specialized organ such as the trichome in some halophytes
[46]. Salt traffic requires transporters in plasma or vacuole membranes. Several transporter genes have been characterized
[48]. The plasma membrane symporters HKT1 in leaves and ATK1 in roots are responsible for pumping K
+ into the cell. Another significant pump is the Salt Overly Sensitive (SOS1) antiporter, which extrudes Na
+ and transports H
+ into the cell
[49]. SOS1 is regulated by phosphorylation of SOS2 protein kinase and Ca
++ sensor SOS3. On the vacuole membrane, Ca
+ regulates Na
+ compartmentalization by the NHX1 antiporter and helps H
+ exit. The other key types of antiporters are V-ATPase and V-PPase, which are responsible for introducing H
+ into the vacuolar membrane
[48].
3. Gene Regulation and Signal Transduction
Complex signaling cascades are induced in response to abiotic stress and are integrated into multistep phosphorelay signaling that includes both hormonal and environmental cues into a common pathway
[50]. The different abiotic stresses can trigger common signaling pathways
[51]. Plant sensing to abiotic stress initiates with membrane or intracellular osmosensors that induce a shift in intracellular Ca
2+ and production of secondary messengers such as inositol phosphate (IP) and ROS, leading to activation of different protein kinases such as calcium-dependent protein kinases (CDPKs), calcium/calmodulin-dependent protein kinases (CCaMKs), mitogen-activated protein kinases (MAPK), or phosphatases that can phosphorylate/dephosphorylate specific transcription factors, which in turn regulate stress-responsive genes
[52]. There is an intricate crosstalk among various plant hormones, namely, abscisic acid (ABA), jasmonic acid (JA), salicylic acid (SA), cytokinins (CK), and ethylene (ET), in coordination with transcription factors such as MYB, bLHL, WRKY, bZIP, NAC, and DREB, to activate or repress stress responses
[53] (
Figure 1).
ABA is a major player in coordinating plant stress responses. Several abiotic stresses, such as drought and high salinity, trigger ABA biosynthesis, which is responsible for key plant processes such as root growth, stomatal closure, and seed germination. The core regulatory pathway of ABA signaling has been identified
[51]. ABA interacts with the regulatory component of the ABA receptor, which in turn inhibits phosphatase type 2C (PP2C), promoting the liberation of SNF1-related protein kinase 2 (SnRK2). The activated SnRK2s phosphorylate downstream effectors to regulate multiple biological processes, such as transcription, RNA processing, epigenetic modification, and flowering time regulation
[54].
JA, a class of fatty acid-derived molecules, also plays an important role in promoting plant response to abiotic stress. It participates in several signal transduction pathways to induce cell protection from the toxic effects of salt stress, drought stress, heavy metal toxicity, micronutrient toxicity, freezing stress, ozone stress, and light stress
[55].
CK acts as a negative regulator of salt and drought tolerance and counteracts ABA and Strigolactones (SLs), a carotenoid-derived phytohormone, which has roles in root development, shoot branching, leaf senescence, plant communication with beneficial microbes, and adaptation to cold, saline, and drought stresses
[56].
Several other molecules are novel plant growth regulators, acting in signaling cascades and cross-talk with regulatory machinery. The precursor of heterocyclic compounds such as chlorophyll, 5-aminolevulinic acid (ALA), improves abiotic stress tolerance, growth, and yield by inducing antioxidant synthesis, nutrient uptake, and photosynthesis
[57]. Citric acid/citrate (CA) has similar effects and relieves heavy metal stress by inducing chelation and precipitation of metal ions
[58]. Nitric oxide (NO) is a redox-active gas that at low concentrations can signal abiotic stress responses in plants by interacting with calcium and hydrogen sulphide
[59]. Melatonin works as an antioxidant against ROS to promote photosynthesis, rooting, growth, and biotic and abiotic stress tolerance, and its membrane receptor has been identified
[60]. Recent studies have revealed the presence of hormone-like peptides and their corresponding receptors, involved in signaling tolerance to abiotic stress
[61]. Interestingly, the SCREW-NUT peptide-receptor pair counteracts the ABA- and pathogen-induced stomata closure
[62].
High-throughput sequencing has revealed an extensive number of miRNAs in plant genomes as key regulators in plant development and mediating biotic and abiotic stress responses
[63]. Outstandingly, another emerging area of stress tolerance is epigenetics, which deals with genetic changes in chromatin functions that are not related to changes in DNA sequence (
Figure 1). Epigenetic modifications, such as DNA methylation and histone acetylation or phosphorylation, prepare chromatin accessibility for transcription machinery, inducing different chromatin conformations for stress-responsive gene expression
[64]. In addition to DNA and histone modifications, multiple long non-coding RNAs (lncRNAs) are the top emerging participants in abiotic stress responses, regulating transcription factors, numerous miRNAs, and stress-responsive mRNAs
[65]. The active changes in epigenetic modifications on stress-responsive genes open or close the chromatin accession to transcriptional or posttranscriptional regulatory machinery. For instance, HDA6 deacetylase is a negative regulator of the PDC1 and ALDH2B7 genes and thus represses acetate biosynthesis. Under drought, the HDA6 enzyme dissociates from the PDC1 and ALDH2B7 genes to activate the acetate pathway. Acetate connects the plant’s ability to adapt to drought stress with metabolism, epigenetic regulation, and JA signaling
[66].
4. Effective Microbes
Rhizosphere microorganisms such as bacteria and fungi promote plant growth and yield but also have an important role in abiotic stress tolerance enhancement in plants (
Figure 1 and
Figure 2c). Plant-microbe interactions signal molecular networks that modulate phytohormone status and gene expression in plants, inducing osmolyte and nutrient accumulation, antioxidant and proton transport machinery, and ion compartmentalization that elicits stress-responsive pathways
[67][68][69]. Plant growth-promoting rhizobacteria (PGPR) can synthesize phytohormones such as indole-3-acetic acid (IAA), an auxin that induces root and shoot growth, or cytokinins that promote cell division and differentiation
[70]. PGPR can also induce plant accumulation of ABA, enhancing the expression of drought tolerance genes that render osmoprotectants and K
+ accumulation, decrease in electrolyte leakage, and increase ROS scavenging capacity
[71]. Plant inoculation with PGPR that contains 1-Aminocycloprpane-1-Carboxylate (ACC) deaminase reduces ethylene and promotes plant growth and yield under salt stress conditions
[72]. Siderophore produced by PGBR facilitates atmospheric nitrogen fixation, phosphate solubilization, and helps nutrient mobilization
[73]. Exopolysaccharides (EPS) from PGPR aid in the formation of biofilms to keep soil moisture and protect plant roots under water scarcity conditions
[74]. Another important group of soil microorganisms that interact with plants are Trichoderma and arbuscular mycorrhizal (AM) fungi that also mitigate abiotic stress by promoting root growth, membrane stability, and water and nutrient uptake
[75][76]. Through triggering phytohormone signaling, these fungi activate plant aquaporins and membrane transporters gene-expression, improve photosynthesis by scavenging ROS, and induce osmolyte accumulation in plant cells to maintain water use efficiency under stress conditions
[76].
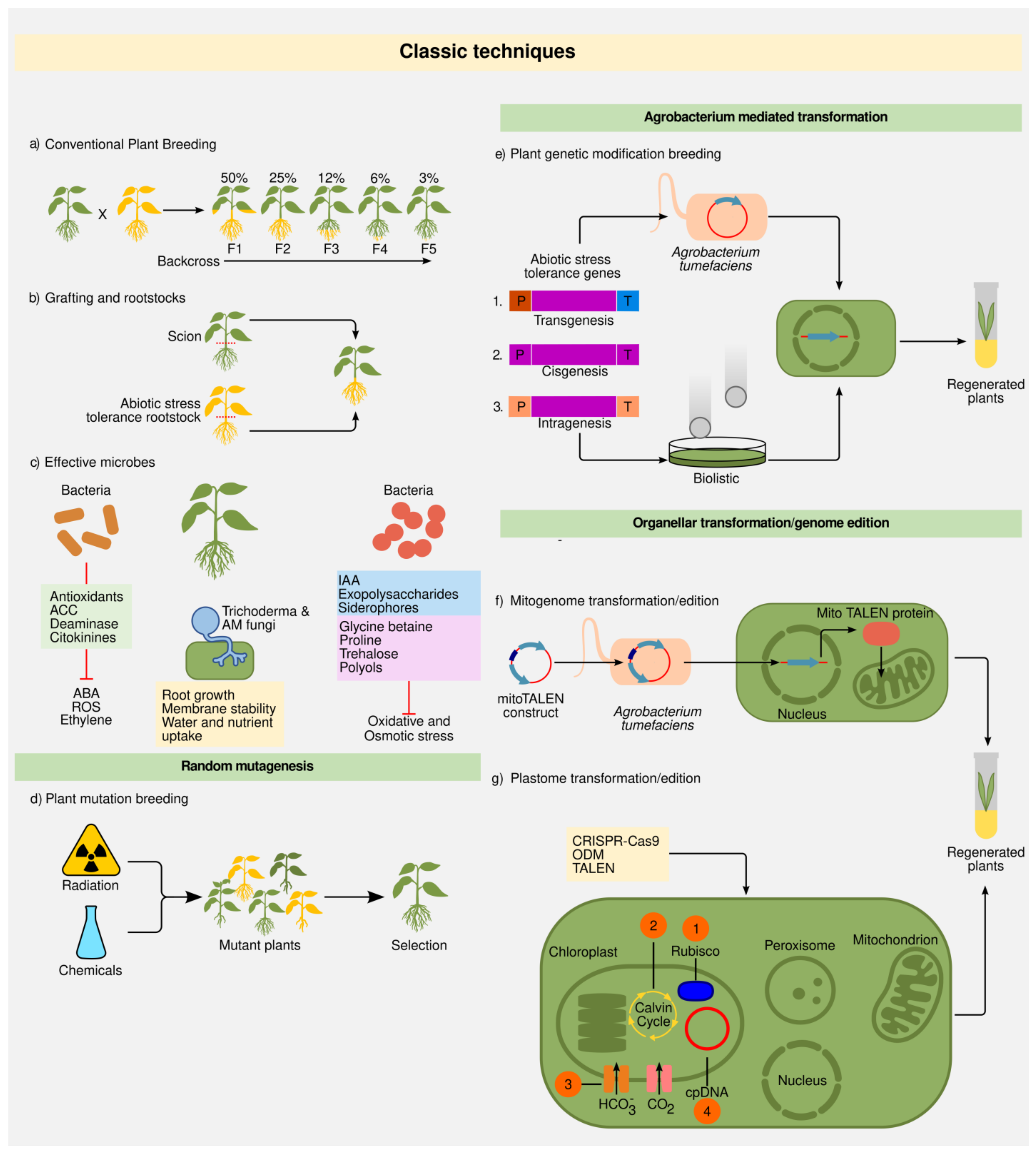
Figure 2. Crop breeding classic techniques and molecular tools. (a) Conventional plant breeding. Pollen from a plant with a desired trait is transferred to the flowers of another variety with other desirable characteristic. Eventually, the desired trait(s) will appear in a new variety of plants through selection. (b) Grafting and roostooks. Grafting is a special type of asexual plant propagation, where a section of a plant (scion) is joined to another plant (rootstock), allowing it to grow as a single plant sharing a unified vascular system. To combine two groups of desired characteristics, usually, both scion and rootstock sources become from different plant varieties. (c) Effective microbes. Plant and microbe interactions involve highly sophisticated symbioses that confer stress tolerance. PGPRs can produce antioxidants, 1-aminocyclopropane-1-carboxylic acid (ACC) deaminase, cytokinins, auxine indoleacetic acid (IAA), exopolysaccharides, siderophores, that inhibit absicic acid (ABA), reactive oxygen species (ROS), and ethylene negative effects. Some bacteria can also produce compounds to increase the solubility and uptake of nutrients from soil or synthetize osmoprotectans that can improve drought responses to plants. Additionally, some fungi help plants by increasing water and nutrient uptake. (d) Plant mutation breeding. It is induced by physical stimulations (X-rays, α and β particles, fast neutrons, and ultraviolet light), or chemical treatments (ethyl methanesulfonate) that generate chromosomal changes that cause random mutations. (e) Agrobacterium tumefaciens and biolistic mediated plant genetic modification breeding. P: promotor; T: teminator. (1) Transgenesis: one or more components such as gene, P, and T that come from sexually incompatible organisms (2) Cisgenesis: all components come from the same original gene (P, gene, and T) isolated from the same species or a sexually compatible organism; (3) Intragenesis: uses one or more components (such as gene, P or T) from different genes of the same species or a sexually compatible organism. (f) Mitogenome transformation/edition. It is carried out through transcription activator-like editing nucleases (TALEN) mediated nuclear transformation. (g) Plastome (plastid genome) transformation/edition. Chloroplast transformation has been used for: (1) Improvement of the catalytic activity of the RUBISCO enzyme, (2) maximize carbon fixation (Calvin cycle) (3) insertion of cyanobacteria transporters, (4) edition focused on different components related to the DNA damage response of the genome, among other applications.
Trichoderma atroviride inoculation diminishes drought effects in maize seedlings by inducing antioxidant machinery
[77]. Moreover, combined inoculation with
Trichoderma and
Pseudomonas minimized the impact of a low watering regime in rice and upregulated genes linked with enzymatic and non-enzymatic antioxidant reactions that helped plant survival under stress
[78]. Recently, a collection of
Trichoderma harzianum mutants obtained by mutagenesis with ethyl methanesulfonate enhanced drought tolerance in Jalapeño pepper plants, phosphorus assimilation, and antagonism against phytopathogenic fungi
[79]. Thus, all these results emphasize that the inoculation of growing plants with microorganism formulations, also known as biofertilizers, has a strategic potential in cultivating crops under abiotic stress conditions.