Magnetic material nanoparticles (MNPs) have been widely used in the detection and treatment of bacterial infections as detection agents and therapeutics. Infections caused by pathogenic bacteria, especially multidrug-resistant bacteria, have become a serious worldwide public health problem. Early diagnosis and treatment can effectively prevent the adverse effects of such infections. Therefore, there is an urgent need to develop effective methods for the early detection, prevention, and treatment of diseases that are caused by bacterial infections.
1. Introduction
Bacterial infections, especially those caused by multidrug-resistant bacteria, have become a global public health concern [1]. The emergence of antimicrobial resistance is largely attributed to the indiscriminate and often abusive use of antimicrobials [2]. It was clinically found that due to the abuse of antibiotics, there is a lack of effective antibacterial drugs to treat bacterial infections [3]. It is estimated that deaths due to infectious diseases that are associated with resistant pathogens will lead to 10 million deaths per year by 2050 [4]. Therefore, there is an urgent need to develop non-antibiotic drugs to treat multidrug-resistant bacterial infections [5].
The early detection and identification of bacterial infections are also major clinical challenges [6]. Moreover, the traditional detection and identification methods are time-consuming and tedious. In order to overcome the shortcomings of traditional antibacterial treatment and detection methods, various metals and metal oxide nanoparticles have been used for bacterial detection and treatment [7]. MNPs have been widely used in the biological field in recent years due to their physical properties, good biocompatibility, and high binding capacity [8][9][10], including in vivo and in vitro bacterial detection and separation imaging [11], as well as the treatment of pathogenic bacteria. For example, magnetic materials were used to synthesize new structures [12] and new magnetic material composite nanoparticles with improved structural stability [13], biological activity [14], and antibacterial properties [15][16][17][18] to realize the separability and recyclability of MNPs [19][20][21].
2. MNPs as Antibacterial Therapies
The discovery of penicillin in 1928 by Alexander Fleming [22] ushered in the “antibiotic era”; penicillin was truly a miracle drug: uniformly fatal infections could be cured. By the mid-1940s after the antibacterial efficacy of penicillin was clinically proven, antibiotics have begun to play a role in various diseases. The discovery of antibiotics has brought effective cures for many diseases to mankind [23]. Yet, as human beings progress, bacteria are constantly evolving. When antibiotics are widely used, they are also constantly losing their antibacterial efficacy, leading to the problem of antibiotic resistance [24][25]. Therefore, the development of new antibiotic therapies is one of the important strategies that are used to combat super-resistant bacteria. In particular, finding new sterilization mechanisms has become a new hot spot in the development of new therapies for the treatment of infections.
Along with the extensive range of exotic NPs applications, the investigation of MNPs in vitro has ushered modern antibacterial studies into an increasingly attractive research area. The great potential of engineered MNPs in the treatment of various resistant bacteria has reduced the threat of deadly bacterial infections [26]. The properties of MNPs allow them to be guided around the body by a magnetic field or into magnetic implants. This opens up the potential to combine various biological materials with nanoparticles, which can then be directed into the body for treatment [27].
2.1. Magnetic Hyperthermia
Magnetic hyperthermia usually uses the principle of electromagnetic waves to generate heat, which acts on soft tissues to achieve therapeutic effects. Generally, it can promote local blood circulation and accelerate the absorption of local inflammation, which can also promote the repair of injured soft tissues. Alumutairi et al. demonstrated that MNP/alternating magnetic field (MNP/AMF) hyperthermia has a synergistic effect with conventional antibiotics within a safe thermal dose threshold
[28]. This was performed by correlating MNP/AMF-induced thermal ablation in an in vitro cell culture setting. For this purpose, varying combinations of MNPs concentration and AMF strength were applied to the culture of
S. aureus biofilm and the change in ambient temperature in the media of
S. aureus biofilm was measured (
Figure 1A). This contributed to the innate immune response of host macrophages to eliminate intracellular bacteria. Abenojar et al. developed thermally responsive magnetic nanocomposites to eliminate pre-formed biofilms
[29]. Loading the hydrogel nanocomposite with MNPs allows for a combined heat treatment after magnetic field (magnetic hyperthermia) stimulation. First, the D-amino acids in the hydrogel nanocomposite inhibited
S. aureus biofilm formation. Then, complete eradication via magnetic hyperthermia occurred, thus realizing an antibacterial treatment (
Figure 4B). The use of the magnetic glycolchitin-based, D-AA-loaded hydrogel—which involves initial treatment for 2 h, followed by additional AMF treatment for 10 min at AC field amplitude of 5 kA/m and a frequency of 380 kHz—showed complete biofilm disruption. NS indicates that the difference in the means of the treatments was not statistically significant at
p > 0.05 (
Figure 1C).
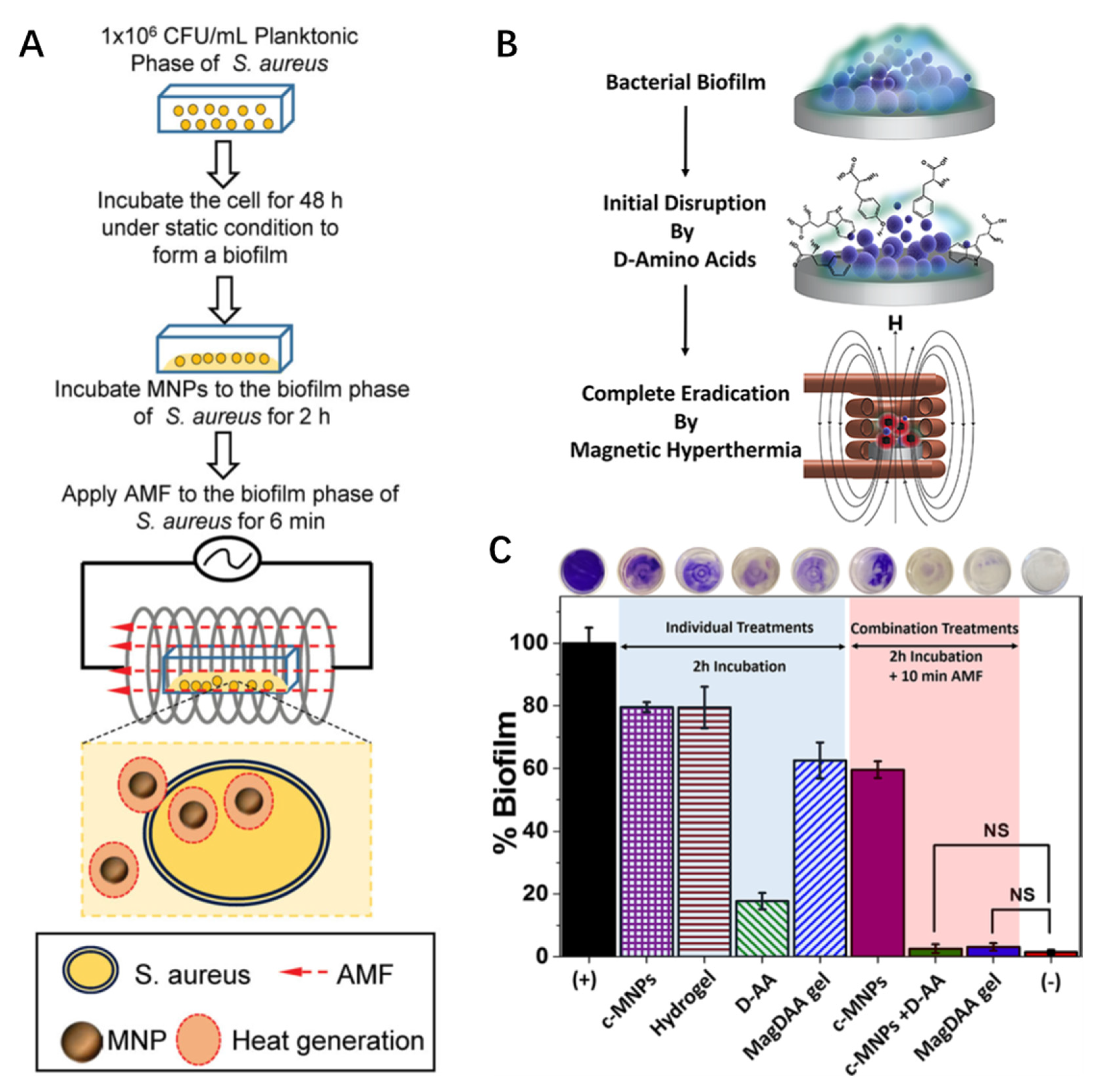
Figure 1. Different schemes of magnetothermal therapy using MNPs: (
A) a schematic diagram of the experimental procedure for the application of MNP/AMF hyperthermia to the biofilm bacteria in vitro (reproduced with permission from
[28]; published by CC BY, 2019); (
B) a schematic diagram on the magnetic hyperthermia for the disruption of bacterial biofilm (reproduced with permission from
[29]; published by the American Chemical Society, 2018); (
C) biofilm disruption activity comparison of the different treatments used in this study and the corresponding crystal violet stained samples of the remaining biofilms after the different treatments (reproduced with permission from
[29]; published by American Chemical Society, 2018).
2.2. Multifunctional MNPs for Theranostics
In order to accurately treat drug-resistant deep-seated bacterial infections, Wang et al. built a polymer–peptide–porphyrin conjugate (PPPC) that can monitor the site of infection in real time based on an in vivo self-assembly strategy and used it to perform precise sonodynamic therapy (SDT) for deep infections
[30]. MRI was used to monitor the porphyrin sonosensitizer (MnTCPP) contained in PPPCs in the infection site in situ. When the concentration of PPPC-1 reaches the minimum inhibitory concentration (MIC), the infected area of drug-resistant bacteria can be exposed to ultrasound irradiation and the bacteria can be killed accurately and efficiently (
Figure 2A).
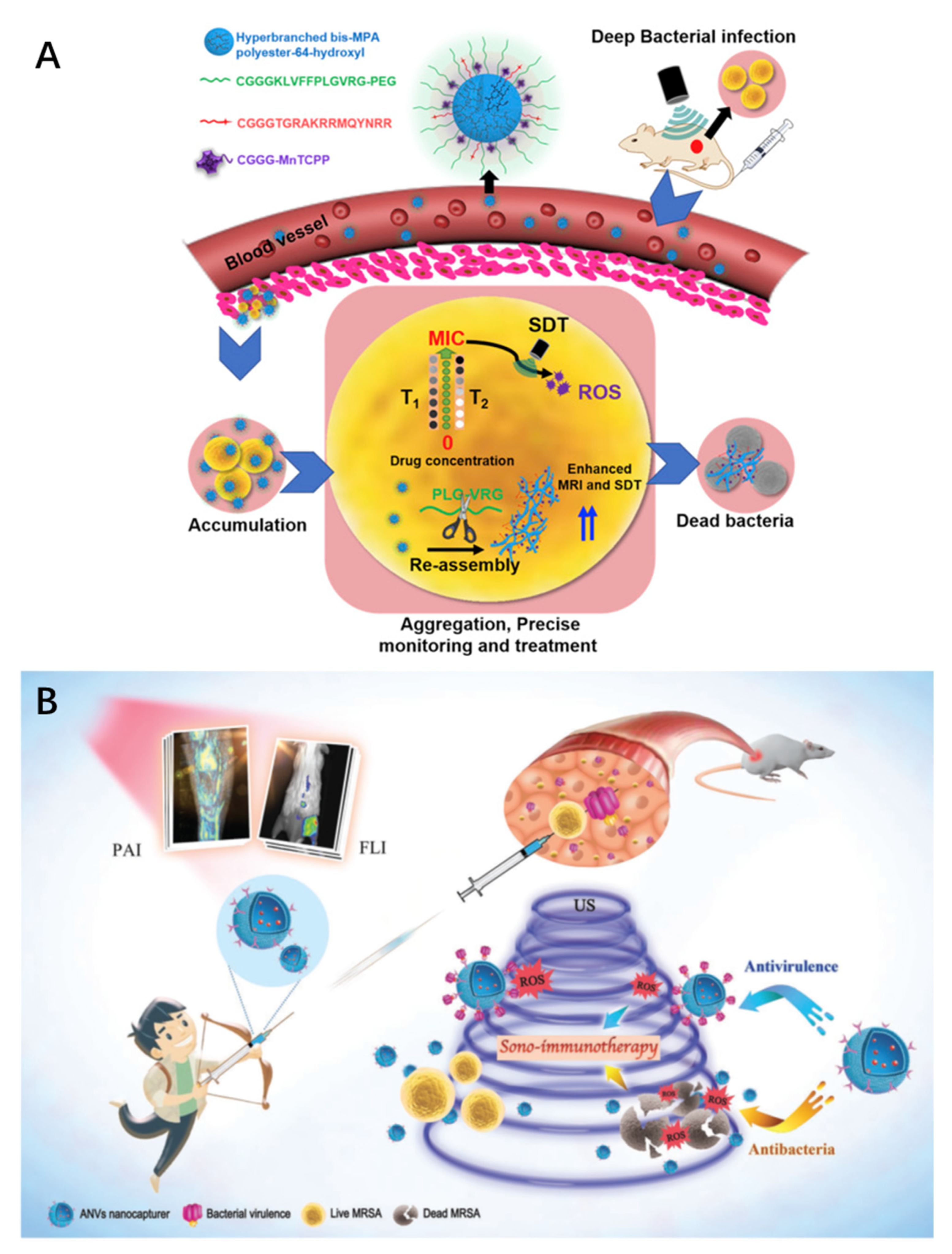
Figure 2. Schematic illustration of experimental procedures: (
A) precise magnetic resonance imaging-guided sonodynamic therapy for drug-resistant bacterial deep infection (reproduced with permission from
[30]; published by Elsevier, 2021); (
B) sono-immunotherapeutic nanocapture to combat multidrug-resistant bacterial infections (reproduced with permission from
[31]; published by John Wiley and Sons, 2021).
3. Summary and Perspectives
MNPs have been extensively studied in bacterial diagnosis and treatment, mainly due to their unique superparamagnetic properties, large specific surface area, and easy surface modification. MNPs also possess excellent antibacterial properties that are mainly driven by magnetic fields and active oxygen generation, which are used to treat bacteria in vitro and in vivo. This article reviewed their application in the detection and treatment of bacterial infections. By also leveraging their unique superparamagnetism, MNPs were used to develop various detection schemes for detecting signal amplification or direct signal transduction. Different detection strategies, including colorimetric, fluorescence, SERS, PAI, and NMR, were developed to detect bacteria. The application of MNPs in the treatment of bacterial infections was also discussed in detail in this review with emphasis on magnetic hyperthermia and theranostics.
MNPs were shown to be superior bacterial detection and therapeutic agents. However, before their clinical application as a diagnostic and therapeutic drug, there are still some challenges to overcome. Hitherto, the utilization of MNPs for the detection of bacteria is still in the proof-of-concept stage, where they were evaluated in facile laboratory samples rather than body fluids. Large-scale tests should be carried out in different complex biological samples to simulate a real environment. Various surface modifications are needed to further improve detection selectivity and material stability, and biocompatibility is an important criterion for clinical applications. Without affecting their antibacterial activity, minimizing the toxicity of MNPs to normal eukaryotic cells is still a very challenging problem. Although there has been a lot of progress in the past few decades on the development of MNPs for bacterial detection, there are still great challenges that need to be resolved before their clinical application, such as the stability of the MNPs, which is essential in the practical application, and non-specifically aggregated particles may be formed in a complex biological system, leading to false positive or false negative signals. In order to overcome these problems, effective surface modification could be used to improve their stability and reduce background signals. Furthermore, the selectivity could be further improved for the detection of bacteria. Suitable surface modification using various strategies to identify agents for the MNPs could be conducted to solve this problem.
Nanomaterials with both photothermal and sonodynamic effects have higher application potential, and their preclinical evaluation needs to be further studied. Despite the therapeutic effects of MNPs summarized in this review, their applications are just in the laboratory stage, which mostly involves in vitro testing. It is common knowledge that various parameters change on transiting from in vitro to in vivo applications. Despite significant progress in MNPs for the treatment of bacterial infections, there are still some issues to be resolved. Toxicity and side effects of the MNPs need to be carefully and extensively investigated to confirm that these nanomaterials are suitable for clinical application. It is necessary to study the toxicity of MNPs, especially improving the bio-compatibility, to reduce non-specific toxicity in vitro and in vivo. Surface modification of MNPs with biocompatible components, such as peptides or polysaccharides, could be the future direction. Finally, the addition of selective recognition agents, such as specific antibiotics or antibodies of identified pathogens, could be explored to further improve the selectivity of the MNPs. Finally, the use of multifunctional MNPs can perform detection and treatment at the same time, realize image-guided treatment, and provide a new direction for the development of nanomedicine. It is ultimately expected that this report will significantly help in developing effective and broad-spectrum magnetic nanomedicines for the treatment of bacterial infections.