Along with cytokines, extracellular vesicles (EVs) released by immune cells in the joint contribute to osteoarthritis (OA) pathogenesis. By high-resolution flow cytometry, we characterized 18 surface markers and 4 proinflammatory cytokines carried by EVs of various sizes in plasma and synovial fluid (SF) from individuals with knee OA, with a primary focus on immune cells that play a major role in OA pathogenesis.
1. Introduction
Autologous plasma products, with cell-free platelet rich plasma (PRP) being chief among them, have been used as intra-articular therapies for osteoarthritis (OA) since the first randomized controlled trials in 2012
[1][2]. However, there is great heterogeneity among products and even PRP preparation methods lack a standardized protocol
[3]. A recent meta-analysis suggested that PRP may be more efficacious than intra-articular hyaluronan (an established OA therapy)
[4]. However, another meta-analysis failed to demonstrate clear evidence for intra-articular PRP efficacy
[5]. On the other hand, in preclinical models, extensive studies of mesenchymal stem cell (MSC)-derived EVs demonstrate beneficial effects in OA
[6][7], such as increased cell proliferation, anti-inflammatory and immunomodulatory effects, and decreased apoptosis
[6]. This evidence supports the emerging understanding that EVs can mediate cell-to-cell communication by transferring their cargo effectors to recipient cells
[8][9][10][11][12]. Taken together, these data suggest that refined plasma products, based on a comprehensive understanding of the therapeutic elements of plasma, could result in safer and more efficacious autologous therapies for arthritis.
EVs could be particularly advantageous as arthritis therapies. First, body fluids contain a high amount of circulating EVs, released by almost all mammalian cells. Second, their surface markers can serve as fingerprints of their parent cell origin and provide a means to isolate EVs from specific cell subsets, such as MSCs
[8][9][10][11][13]. Third, EVs can be isolated from fresh or stored specimens; the use of stored specimens could greatly facilitate subsequent processing to create a defined autologous therapeutic. Thus, to advance to the next generation autologous cell-free biological therapies for OA, there is a critical need to determine the plasma EV surface markers and effects of their cargo effectors (e.g., cytoplasmic proteins, DNA, mRNA, miRNA, small non-coding RNAs, mitochondria, and cytokines) in the context of an individual with OA. In addition, the amount, composition, surface markers, and cargo of EVs can reflect the physiological and pathological condition of the body; therefore, an improved understanding of EVs in OA could produce new biomarkers of disease and elucidate disease pathogenesis.
Evidence for a role of EVs in OA disease pathogenesis exists, believed to be mediated through release of EVs by immune cells in joint tissues and synovial fluid (SF)
[14][15]. However, only limited studies are available that profile EVs in OA biofluids, such as plasma and SF, with a primary focus limited to small EVs (SEVs)
[16][17]. For example, exosomes (a type of SEVs) derived from IL-1β-stimulated synovial fibroblasts induce OA-related gene expression patterns in articular chondrocytes
[18]. In addition to EVs, joint tissue related cytokines play a role in the pathogenesis of OA
[19][20][21][22]. Both EVs and cytokines may originate from multiple joint components including chondrocytes, synovial fibroblasts, subchondral bone, infrapatellar fat pad, tendons, and ligaments
[12][15][23]. The EVs and cytokines derived from these sources can be secreted into SF. Due to potential involvement in OA pathogenesis, EVs and cytokines in SF have the potential to be ‘direct’ biomarkers in the causal pathway of disease
[6][24].
2. Sample Characteristics
A total of 78 study participants with knee OA had available plasma (n = 46) and SF (n = 48) specimens for EV profiling. Of these, a total of 16 participants provided both plasma and SF specimens (age 69 ± 12 years); 30 participants provided only plasma specimens (age 69 ± 8 years); 32 participants provided only SF specimens (age 65 ± 13 years). Age was not significantly different (Kruskal–Wallis test p = 0.4151) between the three cohorts. The sample was 73% older adults (over 60 years of age); and 48% (SF cohort) to 52% (plasma cohort) female. Since the participants were an older adult population with OA, they were taking medicines (such as nonsteroidal anti-inflammatory agents, nutraceuticals-glucosamine and chondroitin sulfate, aspirin, statins, beta-blockers, hormones, anti-depressants) for OA and other common diseases and conditions associated with aging.
3. Multiple Immune Cell-Related EVs Accumulate in SF Compared to Plasma
Recently, we identified three major subsets of plasma EVs in human healthy controls (HCs) using high resolution multicolor flow cytometry: large EVs (LEVs), 1000–6000 nm; medium EVs (MEVs), 100–1000 nm; and SEVs, <100 nm; these major subsets based on size were confirmed using dynamic light scattering
[11]. Similarly, these major subsets of EVs were also identified in plasma (
Figure 1A) and SF (
Figure 1B) of knee OA participants. All 18 tested surface markers of human stem cells and progenitor cells, immune cells, activated pro-inflammatory fibroblasts, epithelial and endothelial cells (
Figure 1C)
[11][25][26][27][28][29][30][31][32][33] were detected on LEVs, MEVs and SEVs from plasma (
Figure 1A) and SF (
Figure 1B) using high resolution multicolor flow cytometry.
Figure 1. Plasma and SF EVs from OA participants carry surface markers from the major hematopoietic cell subsets indicating their cell origins. EVs from plasma and SF of OA participants were profiled with the indicated surface markers by high-resolution multicolor flow cytometry. (A,B) The graphs present the representative color dot plots of all tested surface markers in gated large (LEVs), medium (MEVs) and small (SEVs) EVs from the matched plasma (A) and SF (B) of one OA participant. (C) The table summarizes the tested surface markers and their major expressing cells in human. HSCs: hematopoietic stem cells; ASCs: adipose stem cells; MSCs, mesenchymal stem cells; NK cells: natural killer cells; APCs: antigen presenting cells (including monocytes, macrophages and dendritic cells); HLA-ABC: HLA-A, HLA-B and HLA-C; HLA-DRDPDQ: HLA-DR, -DP and -DQ.
To increase the quantitative informational content of data and simultaneously reduce the data complexity, we analyzed the integrated mean fluorescence intensity (iMFI) of each marker; iMFI is the product of the percentage of the positive population (reflecting frequency of EVs carrying a particular marker) and the MFI of the population (reflecting the mean intensity of the marker in/on the positive EVs)
[34][35]. Compared to the matched plasma EVs, the iMFI in SF EVs was significantly higher for several EV subpopulations: EVs of all sizes with surface markers CD81
+, CD29
+, CD63
+, CD8
+, CD56
+, CD68
+, CD14
+, and major histocompatibility complex (MHC)-class II antigens HLA-DR, -DP and -DQ (HLA-DRDPDQ)
+; MHC-class I antigen HLA-G
+ LEVs and MEVs; CD9
+ MEVs and SEVs; CD19
+ LEVs; and CD235a
+, CD31
+, and MHC-class I antigens HLA-A, HLA-B and HLA-C (HLA-ABC)
+ SEVs (
Figure 2A). The HLA-DRDPDQ
+ LEVs, MEVs and SEVs, reflecting antigen-presenting cells (APCs, including monocytes, macrophages and dendritic cells), activated T cells and pro-inflammatory fibroblasts, were the most enriched EV subpopulations in SF relative to plasma (25-, 50-, and 37-fold higher, respectively), suggesting a major contribution to the SF EV pool from infiltrating immune cells in OA joint tissues
[15][25][36]. In contrast, the CD34
+ MEVs and SEVs, reflecting hematopoietic stem cells (HSCs), progenitor cells, and endothelial cells, were the most significantly enriched EV subpopulations in plasma relative to SF (7.7-, and 7.3-fold higher, respectively). The same surface marker panel yielded similar results in unmatched plasma and SF samples, confirming that the differential profiles between plasma and SF EVs are a general phenomenon in OA (
Figure 2B).
Figure 2. Compared to plasma, multiple immune cell-related EVs are enriched in synovial fluid (SF). EVs from both matched and unmatched plasma and SF of OA participants were profiled with the indicated surface markers by high-resolution multicolor flow cytometry. (A) Comparisons between the matched plasma and SF EVs (n = 16 pairs) were performed using Wilcoxon matched-pairs signed rank test with desired FDR q < 0.05. Volcano plots were generated using −Log 10 (q value) and Log 2 (Fold Changes of iMFI of the individual surface marker in LEVs, MEVs, and SEVs from SF vs Plasma). A positive fold change reflects a higher level of SF EVs relative to plasma EVs; a negative fold change reflects a lower level of SF EVs relative to plasma EVs). (B) These graphs plot the correlation of fold changes (SF ratio to plasma) of iMFI of each surface marker in gated LEVs, MEVs, or SEVs in matched (n = 16 SF-plasma pairs, x axis) and unmatched (n = 32 SF, n = 30 plasma, y axis) SF and plasma EVs.
4. Plasma and SF Correlation of Several Immune Cell-Related EVs and Ratio of Neutrophil-EVs to Lymphocyte-EVs
Although EVs of multiple subpopulations differed in plasma and SF, based on iMFI, several EV subpopulations were significantly correlated between plasma and SF including: positive correlations of CD34+ EVs of all sizes, CD29+ LEVs, and CD15+ and CD19+ MEVs; and negative correlations CD81+ SEVs (Figure 3). In addition, the ratio of neutrophil-EVs to lymphocyte-EVs (which at the cell level represents a pro-inflammatory marker) was also positively correlated between plasma and SF including the ratio of neutrophil-EVs to: EVs related to total lymphocytes (CD15/CD8+4+56+19) and lymphocyte subsets, including T cells (CD15/CD8 and CD15/CD4), NK cells (CD15/CD56), and B cells (CD15/CD19) (Figure 3).
Figure 3. The amount of several immune cell-related EVs and ratio of neutrophil-EVs to lymphocyte-EVs was positively correlated between plasma and SF. EVs from the matched plasma and SF of OA participants (n = 16) were profiled with the indicated surface markers by high-resolution multicolor flow cytometry. Spearman correlation was used for assessing correlations between the matched plasma and SF for the iMFI of individual surface markers and the iMFI ratio of CD15+ neutrophil-related EVs to lymphocyte (CD8+ and CD4+ T cell, CD19+ B cell, and CD56+ NK cell)-related EVs in gated LEVs, MEVs and SEVs. The heat maps were generated using the Spearman correlation coefficient r value with * p < 0.05 and ** p < 0.01.
5. Exo-EV and Endo-EV Cytokines in Plasma and SF
In plasma, based on the same unit volume, the mean concentrations of endo-EV (in lysate of EV pellets) IL-1β and TNF-α were significantly higher, and IL-6 and IFN-γ significantly lower, than the corresponding mean concentrations of exo-EV (in EV-depleted supernatants) cytokines (Figure 4A). Comparing relative concentrations of endo-EV and exo-EV cytokines in SF, the mean concentrations of endo-EV IL-6, TNF-α, and IFN-γ were significantly lower than the corresponding exo-EV cytokines (Figure 4B).
Figure 4. Exo-EV and endo-EV cytokines generally correlated in plasma and SF. (A,B) The concentrations of exo-EV and endo-EV cytokines in plasma and SF of OA participants were measured by multiplex immunoassay. The floating bars (min to max with line at mean) represent the differential concentrations between exo-EV and endo-EV cytokines in plasma (A), n = 46) and SF (B), n = 48). Comparisons between the concentrations of the matched exo-EV and endo-EV cytokines were performed using Wilcoxon matched-pairs signed rank test with desired FDR q < 0.05, and the results are indicated as q value **** < 0.0001. (C,D) EVs from the matched plasma and SF of OA participants (n = 8) were profiled for the indicated intra-vesicle cytokines by high-resolution multicolor flow cytometry. The graphics are representative color dot plots of all tested intra-vesicle cytokines in gated LEVs, MEVs, and SEVs from the matched plasma (C) and SF (D) of one OA participant. (E) The floating bars represent a summary of iMFI (min to max with line at mean) of the tested endo-EV cytokine in gated LEVs, MEVs, or SEVs. Comparisons between the matched plasma and SF EVs (n = 8 each group) were performed using Wilcoxon matched-pairs signed rank test with significant results defined by FDR q < 0.05, asterisks indicate the q value as * < 0.05. (F) Spearman correlation was used for assessing correlations between the concentration of each exo-EV and endo-EV cytokine in plasma (n = 46) and SF (n = 48). The heat maps were generated using the correlation coefficient r value with * p < 0.05, *** p < 0.001, and **** p < 0.0001. (G) Spearman correlation was used for assessing correlations between the matched plasma and SF (n = 8 each group) for the concentration of each exo-EV and endo-EV cytokine. The heat maps were generated using the correlation coefficient r value with * p < 0.05 and ** p < 0.01.
Further analyses based on the same unit volume, EVs from SF were more pro-inflammatory that EVs from plasma. Flow cytometry confirmed that all tested cytokines were carried by EVs of all sizes from OA plasma (Figure 4C) and OA SF (Figure 4D). Among the tested cytokines, TNF-α+ EVs were the most abundant in both plasma and SF (Figure 4E). Moreover, compared to the matched plasma EVs, the iMFI of all tested endo-EV pro-inflammatory cytokines (IL-1β, IL-6, TNF-α and IFN-γ), were higher in SF EVs of all sizes, with statistically significant differences for IL-1β in LEVs, IL-6 in MEVs and SEVs, and IFN-γ in EVs of all sizes (Figure 4E).
Evaluating correlations of endo-EV and exo-EV cytokines within each type of biospecimen (SF and plasma), with the exception of IL-1β in plasma (in low concentration), revealed that concentrations of endo-EV cytokines were significantly positively correlated with the corresponding exo-EV cytokines (Figure 4F). Evaluating correlations of endo-EV and exo-EV cytokines across each type of biospecimen (SF and plasma), indicated that concentrations were significantly positively correlated for: exo-EV IL-1β, IL-6, TNF-α and IFN-γ, and endo-EV IL-6 and TNF-α (Figure 4G).
6. Conclusions
EVs from plasma and SF of OA participants consist of LEVs, MEVs and SEVs that carry cytokines and surface markers related to stem cells and progenitor cells, immune cells, activated pro-inflammatory fibroblasts, epithelial and endothelial cells. Multiple immune cell-derived EV subpopulations were enriched in SF compared with plasma, consistent with OA as an inflammatory arthritis (Figure 5A). The pro-inflammatory phenotype of SF EVs was supported by their pro-inflammatory cytokine cargo (Figure 5A). In contrast, HSC-, progenitor cell-, and endothelial cell-associated EV populations were enriched in plasma relative to SF (Figure 5B). Ratios of neutrophil-EVs to lymphocyte-EVs were positively correlated between plasma and SF (Figure 5C); the ability to derive ratios of neutrophils to lymphocytes from frozen samples by EV profiling can potentially provide a powerful biomarker of OA pathology and other comorbidities, such as cardiovascular disease. EVs related to several types of stem cells, progenitor cells, neutrophils and B cells, and endo-EV pro-inflammatory cytokines IL-6 and TNF-α were highly correlated between SF and plasma (Figure 5D), suggesting plasma EVs have the potential to reflect OA joint inflammation and disease severity. These subpopulations in particular may be direct biomarkers of disease, involved in disease pathogenesis and informing on disease activity.
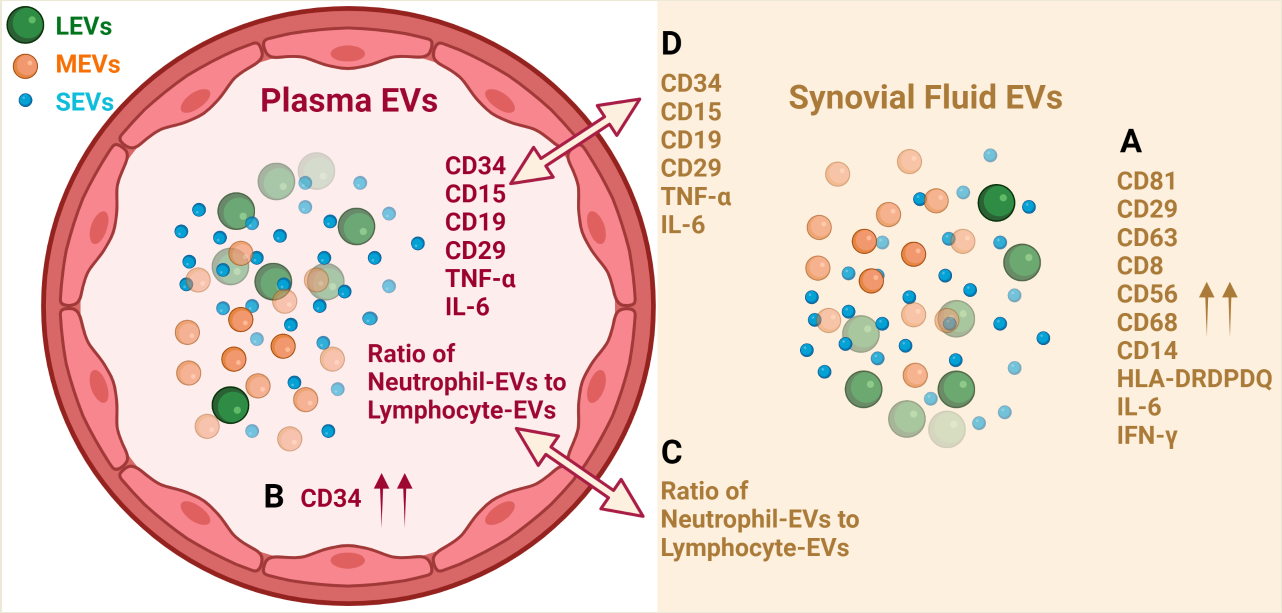